→Fuel cycle: Changed link for reaction comparisons to point directly to the appropriate subsection, instead of the larger section it's contained in. |
Added a bit of extra information on the products of deuterium-tritium fusion reactions (cited) {note: this is my first major information extension, so if it doesn't work well I'm sorry} |
||
Line 1: | Line 1: | ||
{{Short description|Electricity generation through nuclear fusion}} |
|||
{{Infobox general |
|||
{{Distinguish|Fusion of powers}} |
|||
|name = Fusion power |
|||
{{Use mdy dates|cs1-dates=ly|date=June 2020}} |
|||
|image = Sun in X-Ray.png |
|||
[[File:The JET magnetic fusion experiment in 1991.jpg|thumb|upright=1.35|The [[Joint European Torus]] (JET) magnetic fusion experiment in 1991]] |
|||
|caption = The [[Sun]] is a natural fusion reactor. |
|||
}} |
|||
'''Fusion power''' is power generated by [[nuclear fusion]] processes. In fusion reactions two light [[atomic nucleus|atomic nuclei]] fuse together to form a heavier nucleus. In doing so they release a comparatively large amount of energy. |
|||
'''Fusion power''' is a proposed form of [[power generation]] that would generate [[electricity]] by using heat from [[nuclear fusion|nuclear fusion reactions]]. In a fusion process, two lighter [[atomic nucleus|atomic nuclei]] combine to form a heavier nucleus, while releasing energy. Devices designed to harness this energy are known as fusion reactors. Research into fusion reactors began in the 1940s, but as of 2024, no device has reached net power, although net positive reactions have been achieved.<ref name="NYT-209221213">{{cite news |last=Chang |first=Kenneth |title=Scientists Achieve Nuclear Fusion Breakthrough With Blast of 192 Lasers – The advancement by Lawrence Livermore National Laboratory researchers will be built on to further develop fusion energy research. |url=https://www.nytimes.com/2022/12/13/science/nuclear-fusion-energy-breakthrough.html |date=13 December 2022 |work=[[The New York Times]] |access-date=13 December 2022}}</ref><ref name="Ignition">{{Cite news |date=2022-12-13|title=DOE National Laboratory Makes History by Achieving Fusion Ignition|work=US Department of Energy|url=https://www.energy.gov/articles/doe-national-laboratory-makes-history-achieving-fusion-ignition|access-date=2022-12-13}}</ref><ref>{{Cite web |author=Vogt |first1=Adrienne |last2=Hayes |first2=Mike |last3=Nilsen |first3=Ella |last4=Hammond |first4=Elise |date=2022-12-13 |title=December 13, 2022 US officials announce nuclear fusion breakthrough |url=https://www.cnn.com/us/live-news/nuclear-fusion-reaction-us-announcement-12-13-22/index.html |access-date=2022-12-14 |website=CNN |language=en-us}}</ref><ref>{{cite news |last1=Gardner |first1=Timothy |title=US scientists repeat fusion ignition breakthrough for 2nd time |url=https://www.reuters.com/business/energy/us-scientists-repeat-fusion-power-breakthrough-ft-2023-08-06/ |access-date=13 February 2024 |work=Reuters |issue=Dec 13, 2022}}</ref> |
|||
The term is commonly used to refer to potential commercial production of net usable power from a fusion source, similar to the usage of the term "[[steam engine|steam power]]." The leading designs for controlled fusion research use [[magnetic confinement|magnetic]] ([[tokamak]] design) or [[inertial confinement|inertial]] ([[laser]]) [[confinement]] of a [[plasma]], with [[heat]] from the fusion reactions used to operate a [[steam turbine]] which in turn drives [[electrical generator]]s, similar to the process used in [[fossil fuel]] and [[fission power|nuclear fission]] [[power station]]s. |
|||
Fusion processes require fuel and a confined environment with sufficient [[temperature]], [[pressure]], and confinement time to create a [[plasma (physics)|plasma]] in which fusion can occur. The combination of these figures that results in a power-producing system is known as the [[Lawson criterion]]. In stars the most common fuel is [[hydrogen]], and [[gravity]] provides extremely long confinement times that reach the conditions needed for fusion energy production. Proposed fusion reactors generally use heavy hydrogen [[isotope]]s such as [[deuterium]] and [[tritium]] (and especially a [[D+T|mixture of the two]]), which react more easily than [[Protium (isotope)|protium]] (the most common [[isotopes of hydrogen|hydrogen isotope]]) and produce a [[helium]] nucleus and an energized [[neutron]]<ref>{{cite web |title=Fuelling the fusion reaction |url=https://www.iter.org/sci/FusionFuels |website=iter.org |publisher=ITER |access-date=23 June 2024}}</ref>, to allow them to reach the Lawson criterion requirements with less extreme conditions. Most designs aim to heat their fuel to around 100 million kelvins, which presents a major challenge in producing a successful design. [[Tritium]] is extremely rare on earth, having a half life of only ~12.3 years. Consequently, during the operation of envisioned fusion reactors, known as breeder reactors, [[Breeding blanket|helium cooled pebble beds (HCPBs)]] are subjected to [[neutron]] fluxes to generate tritium to complete the fuel cycle.<ref>{{cite journal |last1=Gan |first1=Y |last2=Hernandez |first2=F |last3=et |first3=al |title=Thermal Discrete Element Analysis of EU Solid Breeder Blanket Subjected to Neutron Irradiation |journal=Fusion Science and Technology |date=2017 |volume=66 |issue=1 |pages=83–90 |doi=10.13182/FST13-727 |arxiv=1406.4199 |url=https://hal.science/hal-02356062v1/file/1406.4199.pdf}}</ref> |
|||
Fusion power is believed to have significant safety advantages over current power stations based on nuclear fission. Fusion only takes place under very limited and controlled circumstances (by comparison fission, including catastrophic failure, only requires that there is sufficient fuel within a small enough space). For this reason, a failure of precise control or cessation of fueling quickly shuts down fusion power reactions. There is no possibility of runaway heat build-up or large-scale release of radioactivity, little or no atmospheric [[pollution]], the power source comprises light elements in small quantities which are easily obtained and largely harmless to life, the waste products are short-lived in terms of radioactivity, and there is little overlap with [[nuclear weapons]] technology. |
|||
As a source of power, nuclear fusion has a number of potential advantages compared to [[nuclear fission|fission]]. These include reduced [[radioactivity]] in operation, little high-level [[nuclear waste]], ample fuel supplies (assuming [[tritium]] breeding or some forms of [[Aneutronic fusion|aneutronic fuels]]), and increased safety. However, the necessary combination of temperature, pressure, and duration has proven to be difficult to produce in a practical and economical manner. A second issue that affects common reactions is managing [[neutron]]s that are released during the reaction, which over time [[plasma-facing material|degrade]] many common materials used within the reaction chamber. |
|||
Fusion powered electricity generation was initially believed to be readily achievable, as fission power had been. However the extreme requirements for continuous reactions and [[plasma]] [[containment]] led to projections being extended by several decades, and more than 60 years after the first attempts, commercial power production is still believed to be unlikely before 2040.<ref name="ITERorg">{{cite web |work=The ITER Project |title=Beyond ITER |publisher=Information Services, Princeton Plasma Physics Laboratory |url=http://www.iter.org/Future-beyond.htm |accessdate=5 February 2011 |archiveurl=http://web.archive.org/web/20061107220145/http://www.iter.org/Future-beyond.htm |archivedate=7 November 2006 }} - Projected fusion power timeline</ref> |
|||
Fusion researchers have investigated various confinement concepts. The early emphasis was on three main systems: [[z-pinch]], [[stellarator]], and [[magnetic mirror]]. The current leading designs are the [[tokamak]] and [[inertial confinement fusion|inertial confinement]] (ICF) by [[laser]]. Both designs are under research at very large scales, most notably the [[ITER]] tokamak in France and the [[National Ignition Facility]] (NIF) laser in the United States. Researchers are also studying other designs that may offer less expensive approaches. Among these alternatives, there is increasing interest in [[magnetized target fusion]] and [[inertial electrostatic confinement]], and new variations of the stellarator. |
|||
{{As of|2010|07|}}, the largest experiment by means of magnetic confinement has been the [[Joint European Torus]] (JET). In 1997, JET produced a peak of {{convert|16.1|MW|lk=on}} of fusion power (65% of input power), with fusion power of over {{convert|10|MW|abbr=on}} sustained for over 0.5 sec. In June 2005, its successor, [[ITER]], was announced by the seven parties involved in the project - the United States, China, the European Union (EU), India, Japan, the Russian Federation, and South Korea.<ref name="announcement">[http://www.science.energy.gov/News_Information/News_Room/2006/ITER/ ITER and the Promise of Fusion Energy].</ref> ITER is designed to produce ten times more fusion power than the power put into the [[Plasma_(physics)#Artificial_plasmas|plasma]] over many minutes; for example 50 MW of input power to produce 500 MW of output power. ITER is currently under construction in [[Cadarache]], France. [[DEMO]] is intended as the next generation of research from ITER, and to be the first reactor demonstrating sustained net energy-producing fusion on a commercial scale. It has been proposed to begin construction of DEMO in 2024. |
|||
{{Toclimit}} |
|||
Inertial (laser) confinement, which was for a time seen as more difficult or infeasible, has generally seen less development effort than magnetic approaches. However this approach made a comeback following further innovations, and is being developed at both the [[United States]] [[National Ignition Facility]] as well as the planned [[European Union]] [[HiPER|High Power laser Energy Research]] (HiPER) facility. As of 2010 heating to 3.3 million [[Kelvin]] was achieved<ref name="Bullis">{{cite web |url=http://www.technologyreview.com/blog/energy/24720/ |title=Scientists Overcome Obstacle to Fusion |author=Bullis, Kevin |date=January 28, 2010 |publisher=''[[Technology Review]]'' |accessdate=2010-01-29 }}</ref> and in October 2010 the first integrated ignition test was announced to have been completed successfully with the 192-beam laser system firing over a million joules of ultraviolet laser energy into a capsule filled with hydrogen fuel. Fusion ignition tests are to follow.<ref name="Oct8_10">{{cite web | title = First successful integrated experiment at National Ignition Facility announced | work = General Physics | publisher = PhysOrg.com | date = October 8, 2010 | url = http://www.physorg.com/news205740709.html | accessdate = 2010-10-09 }}</ref> |
|||
== |
== Background == |
||
{{main|Nuclear fusion}} |
|||
[[Image:fusion rxnrate.svg|right|300px|thumb|The fusion reaction rate increases rapidly with temperature until it maximizes and then gradually drops off. The deuterium-tritium fusion rate peaks at a lower temperature (about 70 keV, or 800 million kelvins) and at a higher value than other reactions commonly considered for fusion energy.]] |
|||
[[File:Sun in X-Ray.png|thumb|upright=1|The [[Sun]], like other [[star]]s, is a natural fusion reactor, where [[stellar nucleosynthesis]] transforms lighter elements into heavier elements with the release of energy.]] |
|||
[[File:Binding energy curve - common isotopes.svg|thumb|upright=2|[[Binding energy]] for different [[atomic nucleus|atomic nuclei]]. Iron-56 has the highest, making it the most stable. Nuclei to the left are likely to release energy when they fuse ([[nuclear fusion|fusion]]); those to the far right are likely to be unstable and release energy when they split ([[nuclear fission|fission]]).]] |
|||
=== Mechanism === |
|||
The basic concept behind any fusion reaction is to bring two or more nuclei close enough together so that the [[strong interaction|residual strong force]] (nuclear force) in their nuclei will pull them together into one larger nucleus. If two light nuclei fuse, they will generally form a single nucleus with a slightly smaller mass than the sum of their original masses (though this is not always the case). The difference in mass is released as energy according to [[Albert Einstein]]'s [[mass-energy equivalence]] formula ''E'' = ''mc''<sup>2</sup>. If the input nuclei are sufficiently massive, the resulting fusion product will be heavier than the sum of the reactants' original masses, in which case the reaction requires an external source of energy. The dividing line between "light" and "heavy" is [[iron]]-56. Above this atomic mass, energy will generally be released by [[nuclear fission]] reactions; below it, by fusion |
|||
Fusion reactions occur when two or more atomic nuclei come close enough for long enough that the [[nuclear force]] pulling them together exceeds the [[electrostatic force]] pushing them apart, fusing them into heavier nuclei. For nuclei heavier than [[iron-56]], the reaction is [[endothermic]], requiring an input of energy.<ref>{{cite web|url=http://hyperphysics.phy-astr.gsu.edu/hbase/nucene/nucbin.html#c2|title=Fission and fusion can yield energy|publisher=Hyperphysics.phy-astr.gsu.edu|access-date=30 October 2014}}</ref> The heavy nuclei bigger than iron have many more protons resulting in a greater repulsive force. For nuclei lighter than iron-56, the reaction is [[exothermic]], releasing energy when they fuse. Since hydrogen has a single [[proton]] in its nucleus, it requires the least effort to attain fusion, and yields the most net energy output. Also since it has one electron, hydrogen is the easiest fuel to fully ionize. |
|||
.<ref>{{Cite web|url=http://hyperphysics.phy-astr.gsu.edu/hbase/nucene/nucbin.html#c2|title=Fission and fusion can yield energy}}</ref> |
|||
The repulsive electrostatic interaction between nuclei operates across larger distances than the strong force, which has a range of roughly one [[femtometer]]—the diameter of a proton or neutron. The fuel atoms must be supplied enough kinetic energy to approach one another closely enough for the strong force to overcome the electrostatic repulsion in order to initiate fusion. The "[[Coulomb barrier]]" is the quantity of [[kinetic energy]] required to move the fuel atoms near enough. Atoms can be heated to extremely high temperatures or accelerated in a particle accelerator to produce this energy. |
|||
Fusion between the nuclei is opposed by their shared electrical charge, specifically the net positive charge of the [[proton]]s in the nucleus. To overcome this [[electrostatic force]], or "[[Coulomb barrier]]", some external source of energy must be supplied. The easiest way to do this is to heat the atoms, which has the side effect of stripping the [[electron]]s from the atoms and leaving them as bare nuclei. In most experiments the nuclei and electrons are left in a fluid known as a [[plasma (physics)|plasma]]. The temperatures required to provide the nuclei with enough energy to overcome their repulsion is a function of the total charge, so [[hydrogen]], which has the smallest nuclear charge therefore reacts at the lowest temperature. [[Helium]] has an extremely low mass per nucleon and therefore is energetically favoured as a fusion product. As a consequence, most [[Nuclear fusion#Important reactions|fusion reactions]] combine isotopes of hydrogen ("[[Hydrogen atom|protium]]", [[deuterium]], or [[tritium]]) to form isotopes of helium ({{SimpleNuclide|Helium|3}} or {{SimpleNuclide|Helium|4}}). |
|||
An atom loses its electrons once it is heated past its [[ionization energy]]. An [[ion]] is the name for the resultant bare nucleus. The result of this ionization is plasma, which is a heated cloud of ions and free electrons that were formerly bound to them. Plasmas are [[electrically conducting]] and magnetically controlled because the charges are separated. This is used by several fusion devices to confine the hot particles. |
|||
The reaction '''[[cross section (physics)|cross section]]''', denoted σ, is a measure of the probability of a fusion reaction as a function of the relative velocity of the two reactant nuclei. If the reactants have a distribution of velocities, as is the case in a thermal distribution within a plasma, then it is useful to perform an average over the distributions of the product of cross section and velocity. The reaction rate (fusions per volume per time) is <σv> times the product of the reactant [[number density|number densities]]: |
|||
=== Cross section === |
|||
: <big>ƒ = (½n)<sup>2</sup> <σv> </big>(for one reactant) |
|||
[[File:Fusion rxnrate.svg|right|upright=1.5|thumb|The fusion reaction rate increases rapidly with temperature until it maximizes and then gradually drops off. The deuterium-tritium (D-T) fusion rate peaks at a lower temperature (about 70 keV, or 800 million kelvin) and at a higher value than other reactions commonly considered for fusion energy.]] |
|||
: <big>ƒ = n<sub>1</sub>n<sub>2</sub> <σv> </big>(for two reactants) |
|||
A reaction's [[cross section (physics)|cross section]], denoted σ, measures the probability that a fusion reaction will happen. This depends on the relative velocity of the two nuclei. Higher relative velocities generally increase the probability, but the probability begins to decrease again at very high energies.<ref name="osti.gov">{{cite report |url=https://digital.library.unt.edu/ark:/67531/metadc872035/ |title=Fusion cross sections and reactivities |last1=Miley |first1=G. H. |last2=Towner |first2=H. |date=1974-06-17 |doi=10.2172/4014032 |osti=4014032 |last3=Ivich |first3=N. |type=Technical Report |via=Osti.gov|doi-access=free }}</ref> |
|||
<σv> increases from virtually zero at room temperatures up to meaningful magnitudes at temperatures of {{convert|10|-|100|keV|abbr=on|lk=on}}. The significance of <σv> as a function of temperature in a device with a particular energy [[confinement time]] is found by considering the [[Lawson criterion]]. |
|||
In a plasma, particle velocity can be characterized using a [[probability distribution]]. If the plasma is [[Thermalisation|thermalized]], the distribution looks like a [[Gaussian curve]], or [[Maxwell–Boltzmann distribution]]. In this case, it is useful to use the average particle cross section over the velocity distribution. This is entered into the volumetric fusion rate:<ref name="Lawson">{{cite journal |last=Lawson |first=J. D. |date=1 December 1956 |title=Some Criteria for a Power Producing Thermonuclear Reactor |journal=Proceedings of the Physical Society. Section B |publisher=IOP Publishing |volume=70 |issue=1 |pages=6–10 |doi=10.1088/0370-1301/70/1/303 |bibcode=1957PPSB...70....6L |issn=0370-1301}}</ref> |
|||
Perhaps the three most widely considered fuel cycles are based on the D-T, D-D, and p-{{SimpleNuclide|Boron|11}} reactions.{{Citation needed|date=October 2009}} Other fuel cycles (D-{{SimpleNuclide|Helium|3}} and {{SimpleNuclide|Helium|3}}-{{SimpleNuclide|Helium|3}}) would require a supply of <sup>3</sup>He, either from other nuclear reactions or from extraterrestrial sources, such as the surface of the moon or the atmospheres of the gas giant planets. The details of the calculations comparing these reactions can be found [[Nuclear fusion#Criteria and candidates for terrestrial reactions|here]]. |
|||
:<math>P_\text{fusion} = n_A n_B \langle \sigma v_{A,B} \rangle E_\text{fusion}</math> |
|||
===D-T fuel cycle=== |
|||
[[Image:Deuterium-tritium fusion.svg|thumb|Diagram of the D-T reaction]] |
|||
The easiest (according to the [[Lawson criterion]]) and most immediately promising nuclear reaction to be used for fusion power is: |
|||
where: |
|||
:{{Nuclide|Deuterium|link=yes}} + {{Nuclide|Tritium|link=yes}} → {{Nuclide|Helium|link=yes}} + {{SubatomicParticle|10neutron|link=yes}} |
|||
* <math>P_\text{fusion}</math> is the energy made by fusion, per time and volume |
|||
Hydrogen-2 ([[Deuterium]]) is a naturally occurring [[isotope]] of hydrogen and as such is universally available. The large mass ratio of the hydrogen isotopes makes the separation rather easy compared to the difficult [[uranium enrichment]] process. Hydrogen-3 (Tritium) is also an isotope of hydrogen, but it occurs naturally in only negligible amounts due to its [[Beta decay|radioactive]] [[half-life]] of 12.32 years. Consequently, the deuterium-tritium fuel cycle requires the [[breeder reactor|breeding]] of [[tritium]] from [[lithium]] using one of the following reactions: |
|||
* ''n'' is the number density of species A or B, of the particles in the volume |
|||
* <math>\langle \sigma v_{A,B} \rangle</math> is the cross section of that reaction, average over all the velocities of the two species ''v'' |
|||
* <math>E_\text{fusion}</math> is the energy released by that fusion reaction. |
|||
=== Lawson criterion === |
|||
:{{SubatomicParticle|10neutron}} + {{Nuclide|Lithium|6}} → {{Nuclide|Tritium}} + {{Nuclide|Helium}} |
|||
The [[Lawson criterion]] considers the energy balance between the energy produced in fusion reactions to the energy being lost to the environment. In order to generate usable energy, a system would have to produce more energy than it loses. Lawson assumed an [[First law of thermodynamics|energy balance]], shown below.<ref name="Lawson"/> |
|||
:{{SubatomicParticle|10neutron}} + {{Nuclide|Lithium|7}} → {{Nuclide|Tritium}} + {{Nuclide|Helium}} + {{SubatomicParticle|10neutron}} |
|||
:<math>P_\text{out} = \eta_\text{capture}\left(P_\text{fusion} - P_\text{conduction} - P_\text{radiation}\right)</math> |
|||
The reactant neutron is supplied by the D-T fusion reaction shown above, the one that also produces the useful energy. The reaction with <sup>6</sup>Li is [[exothermic reaction|exothermic]], providing a small energy gain for the reactor. The reaction with <sup>7</sup>Li is [[endothermic reaction|endothermic]] but does not consume the neutron. At least some <sup>7</sup>Li reactions are required to replace the neutrons lost by reactions with other elements. Most reactor designs use the naturally occurring mix of lithium isotopes. However, the supply of lithium is relatively limited with other applications such as Li-ion batteries increasing its demand. |
|||
where: |
|||
* <math>P_\text{out}</math> is the net power from fusion |
|||
* <math>\eta_\text{capture}</math> is the efficiency of capturing the output of the fusion |
|||
* <math>P_\text{fusion}</math> is the rate of energy generated by the fusion reactions |
|||
* <math>P_\text{conduction}</math> is the conduction losses as energetic mass leaves the plasma |
|||
* <math>P_\text{radiation}</math> is the radiation losses as energy leaves as light. |
|||
The rate of fusion, and thus P<sub>fusion</sub>, depends on the temperature and density of the plasma. The plasma loses energy through [[Thermal conduction|conduction]] and [[radiation]].<ref name="Lawson"/> Conduction occurs when [[ion]]s, [[electron]]s, or [[neutral particle|neutrals]] impact other substances, typically a surface of the device, and transfer a portion of their kinetic energy to the other atoms. The rate of conduction is also based on the temperature and density. Radiation is energy that leaves the cloud as light. Radiation also increases with temperature as well as the mass of the ions. Fusion power systems must operate in a region where the rate of fusion is higher than the losses. |
|||
Several drawbacks are commonly attributed to D-T fusion power: |
|||
# It produces substantial amounts of neutrons that result in [[induced radioactivity]] within the reactor structure.<ref name="Thinkquest">{{Cite web| publisher= | url=http://library.thinkquest.org/17940/texts/fusion_dt/fusion_dt.html | title= Thinkquest: D-T reaction | first= | last= | date | accessdate=12 June 2010 | postscript= <!--None-->}}</ref> |
|||
# Only about 20% of the fusion energy yield appears in the form of charged particles (the rest neutrons), which limits the extent to which direct energy conversion techniques might be applied.{{Citation needed|date=October 2009}} |
|||
# The use of D-T fusion power depends on lithium resources, which are less abundant than deuterium resources. However, lithium is relatively abundant on earth.<ref name="Thinkquest" /> |
|||
# It requires the handling of the radioisotope tritium. Similar to hydrogen, tritium is difficult to contain and may leak from reactors in some quantity. Some estimates suggest that this would represent a fairly large environmental release of radioactivity.<ref>{{Cite web|url=http://www.world-nuclear.org/info/inf66.html|title=Nuclear Fusion Power, Assessing fusion power}}</ref> |
|||
=== Triple product: density, temperature, time === |
|||
The [[neutron flux]] expected in a commercial D-T fusion reactor is about 100 times that of current fission power reactors, posing problems for material design. Design of suitable materials is under way but their actual use in a reactor is not proposed until the generation after [[ITER]]. After a single series of D-T tests at [[Joint European Torus|JET]], the largest fusion reactor yet to use this fuel, the vacuum vessel was sufficiently radioactive that remote handling needed to be used for the year following the tests. |
|||
[[File:Fusion Triples 2021.png|thumb|upright=2|alt=Fusion trapping (left) against temperature (bottom) for various fusion approaches as of 2021, assuming DT fuel. |Fusion trapping (left) against temperature (bottom) for various fusion approaches as of 2021, assuming DT fuel<ref>Wurzel, Samuel E., and Scott C. Hsu. "Progress toward fusion energy breakeven and gain as measured against the Lawson criterion." arXiv preprint arXiv:2105.10954 (2021).</ref>{{clarify|reason=What are the shaded bands in the upper right corner in blue, green, yellow and red? Need to explain their meaning|date=June 2022}}]] |
|||
The [[Lawson criterion]] argues that a machine holding a thermalized and quasi-[[Neutral particle|neutral]] plasma has to generate enough energy to overcome its energy losses. The amount of energy released in a given volume is a function of the temperature, and thus the reaction rate on a per-particle basis, the density of particles within that volume, and finally the confinement time, the length of time that energy stays within the volume.<ref name="Lawson"/><ref>{{cite web |url=http://www.efda.org/2013/02/triple-product/ |title=Lawson's three criteria |publisher=EFDA |date=February 25, 2013 |access-date=2014-08-24 |archive-url=https://web.archive.org/web/20140911210243/http://www.efda.org/2013/02/triple-product/ |archive-date=2014-09-11 |url-status=dead }}</ref> This is known as the "triple product": the plasma density, temperature, and confinement time.<ref>{{cite web |url=http://www.efda.org/glossary/triple-product/ |title=Triple product |publisher=EFDA |date=2014-06-20 |access-date=2014-08-24 |archive-url=https://web.archive.org/web/20140911205015/http://www.efda.org/glossary/triple-product/ |archive-date=2014-09-11 |url-status=dead }}</ref> |
|||
In magnetic confinement, the density is low, on the order of a "good vacuum". For instance, in the [[ITER]] device the fuel density is about {{nowrap|1.0 × 10<sup>19</sup> m<sup>−3</sup>}}, which is about one-millionth atmospheric density.<ref>{{cite web |url=https://accelconf.web.cern.ch/e06/TALKS/FRYCPA01_TALK.PDF |title=ITER and the International ITER and the International Scientific Collaboration |first=Stefano |last=Chiocchio}}</ref> This means that the temperature and/or confinement time must increase. Fusion-relevant temperatures have been achieved using a variety of heating methods that were developed in the early 1970s. In modern machines, {{as of|2019|lc=yes}}, the major remaining issue was the confinement time. Plasmas in strong magnetic fields are subject to a number of inherent instabilities, which must be suppressed to reach useful durations. One way to do this is to simply make the reactor volume larger, which reduces the rate of leakage due to [[classical diffusion]]. This is why ITER is so large. |
|||
In a production setting, the neutrons would be used to react with [[lithium]] in order to create more tritium. This also deposits the energy of the neutrons in the lithium, which would then be cooled to remove this energy and drive electrical production. This reaction protects the outer portions of the reactor from the neutron flux. Newer designs, the advanced tokamak in particular, also use lithium inside the reactor core as a key element of the design. The plasma interacts directly with the lithium, preventing a problem known as "recycling". The advantage of this layout was demonstrated in the [[Lithium Tokamak Experiment]]. |
|||
In contrast, inertial confinement systems approach useful triple product values via higher density, and have short confinement intervals. In [[National Ignition Facility|NIF]], the initial frozen hydrogen fuel load has a density less than water that is increased to about 100 times the density of lead. In these conditions, the rate of fusion is so high that the fuel fuses in the microseconds it takes for the heat generated by the reactions to blow the fuel apart. Although NIF is also large, this is a function of its "driver" design, not inherent to the fusion process. |
|||
=== Energy capture === |
|||
Multiple approaches have been proposed to capture the energy that fusion produces. The simplest is to heat a fluid. The commonly targeted D-T reaction releases much of its energy as fast-moving neutrons. Electrically neutral, the neutron is unaffected by the confinement scheme. In most designs, it is captured in a thick "blanket" of [[lithium]] surrounding the reactor core. When struck by a high-energy neutron, the blanket heats up. It is then actively cooled with a working fluid that drives a turbine to produce power. |
|||
Another design proposed to use the neutrons to breed fission fuel in a blanket of [[nuclear waste]], a concept known as a [[fission-fusion hybrid]]. In these systems, the power output is enhanced by the fission events, and power is extracted using systems like those in conventional fission reactors.<ref>{{cite web|url=https://life.llnl.gov/ |title=Laser Inertial Fusion Energy |publisher=Life.llnl.gov |access-date=2014-08-24 |url-status=dead |archive-url=https://web.archive.org/web/20140915170021/https://life.llnl.gov/ |archive-date=2014-09-15 }}</ref> |
|||
Designs that use other fuels, notably the proton-boron [[aneutronic fusion]] reaction, release much more of their energy in the form of charged particles. In these cases, power extraction systems based on the movement of these charges are possible. [[Direct energy conversion]] was developed at [[Lawrence Livermore National Laboratory]] (LLNL) in the 1980s as a method to maintain a voltage directly using fusion reaction products. This has demonstrated energy capture efficiency of 48 percent.<ref name="ReferenceA">{{cite journal | last1=Barr | first1=W. L. | last2=Moir | first2=R. W. | last3=Hamilton | first3=G. W. | title=Experimental results from a beam direct converter at 100 kV | journal=Journal of Fusion Energy | publisher=Springer Science and Business Media LLC | volume=2 | issue=2 | year=1982 | issn=0164-0313 | doi=10.1007/bf01054580 | pages=131–143| bibcode=1982JFuE....2..131B | s2cid=120604056 }}</ref> |
|||
== Plasma behavior == |
|||
Plasma is an ionized gas that conducts electricity.<ref>{{Cite book |last=Fitzpatrick |first=Richard |title=Plasma physics: an introduction |date=2014 |isbn=978-1466594265 |location=Boca Raton, Florida |publisher=CRC Press, Taylor & Francis Group |language=en-us |oclc=900866248}}</ref> In bulk, it is modeled using [[magnetohydrodynamics]], which is a combination of the [[Navier–Stokes equations]] governing fluids and [[Maxwell's equations]] governing how [[magnetic field|magnetic]] and [[electric field]]s behave.<ref>{{cite journal |last1=Alfvén |first1=H. |year=1942 |title=Existence of electromagnetic-hydrodynamic waves |journal=Nature |volume=150 |issue=3805 |pages=405–406 |bibcode=1942Natur.150..405A |doi=10.1038/150405d0 |s2cid=4072220}}</ref> Fusion exploits several plasma properties, including: |
|||
* Self-organizing plasma conducts electric and magnetic fields. Its motions generate fields that can in turn contain it.<ref>{{cite journal |last1=Tuszewski |first1=M. |year=1988 |title=Field reversed configurations |url=https://zenodo.org/record/1235740 |journal=Nuclear Fusion |type=Submitted manuscript |volume=28 |issue=11 |pages=2033–2092 |doi=10.1088/0029-5515/28/11/008 |s2cid=122791237|doi-access=free }}</ref> |
|||
* [[Diamagnetism|Diamagnetic plasma]] can generate its own internal magnetic field. This can reject an externally applied magnetic field, making it diamagnetic.<ref>{{Cite journal |last1=Sijoy |first1=C. D. |last2=Chaturvedi |first2=Shashank |date=2012 |title=An Eulerian MHD model for the analysis of magnetic flux compression by expanding diamagnetic fusion plasma sphere |url=http://dx.doi.org/10.1016/j.fusengdes.2011.10.012 |journal=Fusion Engineering and Design |volume=87 |issue=2 |pages=104–117 |doi=10.1016/j.fusengdes.2011.10.012 |bibcode=2012FusED..87..104S |issn=0920-3796}}</ref> |
|||
* [[Magnetic mirror]]s can reflect plasma when it moves from a low to high density field.<ref>{{Cite book |last=Post |first=R. F. |title=Proceedings of the second United Nations International Conference on the Peaceful Uses of Atomic Energy held in Geneva 1 September – 13 September 1958 |volume=32 |date=1958 |publisher=United Nations |editor-last=United Nations International Conference on the Peaceful Uses of Atomic Energy |location=Geneva, Switzerland |language=en |oclc=643589395}}</ref><sup>:24</sup> |
|||
== Methods == |
|||
[[File:Chart of Fusion Approaches.png|thumb|upright=2|Approaches to fusion, in color coded families: Pinch Family (orange), Mirror Family (red), Cusp Systems (violet), Tokamaks & Stellarators (Green), Plasma Structures (gray), Inertial Electrostatic Confinement (dark yellow), Inertial Confinement Fusion (ICF, blue), Plasma Jet Magneto Inertial Fusion (PJMIF, dark pink).]] |
|||
=== Magnetic confinement === |
|||
{{Main|Magnetic confinement fusion}} |
|||
* [[Tokamak]]: the most well-developed and well-funded approach. This method drives hot plasma around in a magnetically confined [[torus]], with an internal current. When completed, ITER will become the world's largest tokamak. As of September 2018 an estimated 226 experimental tokamaks were either planned, decommissioned or operating (50) worldwide.<ref>{{Cite web|title=All-the-Worlds-Tokamaks|url=http://www.tokamak.info/|access-date=2020-10-11|website=www.tokamak.info}}</ref> |
|||
* [[Spherical tokamak]]: also known as spherical torus. A variation on the tokamak with a spherical shape. |
|||
* [[Stellarator]]: Twisted rings of hot plasma. The stellarator attempts to create a natural twisted plasma path, using external magnets. Stellarators were developed by [[Lyman Spitzer]] in 1950 and evolved into four designs: Torsatron, Heliotron, Heliac and Helias. One example is [[Wendelstein 7-X]], a German device. It is the world's largest stellarator.<ref>{{Cite web|title=The first plasma: the Wendelstein 7-X fusion device is now in operation|url=https://www.ipp.mpg.de/3984226/12_15|access-date=2020-10-11|website=www.ipp.mpg.de|language=en}}</ref> |
|||
* Internal rings: Stellarators create a twisted plasma using external magnets, while tokamaks do so using a current induced in the plasma. Several classes of designs provide this twist using conductors inside the plasma. Early calculations showed that collisions between the plasma and the supports for the conductors would remove energy faster than fusion reactions could replace it. Modern variations, including the [[Levitated dipole|Levitated Dipole Experiment (LDX)]], use a solid superconducting torus that is magnetically levitated inside the reactor chamber.<ref>{{Cite web|last=Chandler|first=David|title=MIT tests unique approach to fusion power|url=https://news.mit.edu/2008/ldx-tt0319|access-date=2020-10-11|website=MIT News {{!}} Massachusetts Institute of Technology|date=March 19, 2008 |language=en}}</ref> |
|||
* [[Magnetic mirror]]: Developed by [[Richard F. Post]] and teams at Lawrence Livermore National Laboratory ([[LLNL]]) in the 1960s.<ref name="Post 99–111">{{Citation|last=Post|first=R. F.|title=Mirror systems: fuel cycles, loss reduction and energy recovery|date=1970-01-01|url=https://www.icevirtuallibrary.com/doi/abs/10.1680/nfr.44661.0007|work=Nuclear fusion reactors|pages=99–111|series=Conference Proceedings|publisher=Thomas Telford Publishing|doi=10.1680/nfr.44661|isbn=978-0727744661|access-date=2020-10-11}}</ref> Magnetic mirrors reflect plasma back and forth in a line. Variations included the [[Tandem Mirror Experiment|Tandem Mirror]], magnetic bottle and the [[biconic cusp]].<ref>{{Cite book|last1=Berowitz|first1=J. L |title=Proceedings of the second United Nations International Conference on the Peaceful Uses of Atomic Energy |volume=31|last2=Grad|first2=H.|last3=Rubin|first3=H.|date=1958|publisher=United Nations|location=Geneva|language=en|oclc=840480538}}</ref> A series of mirror machines were built by the US government in the 1970s and 1980s, principally at LLNL.<ref>{{cite journal | last1=Bagryansky | first1=P. A. | last2=Shalashov | first2=A. G. | last3=Gospodchikov | first3=E. D. | last4=Lizunov | first4=A. A. | last5=Maximov | first5=V. V. | last6=Prikhodko | first6=V. V. | last7=Soldatkina | first7=E. I. | last8=Solomakhin | first8=A. L. | last9=Yakovlev | first9=D. V. | title=Threefold Increase of the Bulk Electron Temperature of Plasma Discharges in a Magnetic Mirror Device | journal=Physical Review Letters | volume=114 | issue=20 | date=18 May 2015 | issn=0031-9007 | doi=10.1103/physrevlett.114.205001 | pmid=26047233 | page=205001| arxiv=1411.6288 | bibcode=2015PhRvL.114t5001B | s2cid=118484958 }}</ref> However, calculations in the 1970s estimated it was unlikely these would ever be commercially useful. |
|||
* [[Bumpy torus]]: A number of magnetic mirrors are arranged end-to-end in a toroidal ring. Any fuel ions that leak out of one are confined in a neighboring mirror, permitting the plasma pressure to be raised arbitrarily high without loss. An experimental facility, the ELMO Bumpy Torus or EBT was built and tested at [[Oak Ridge National Laboratory]] (ORNL) in the 1970s. |
|||
* [[Field-reversed configuration]]: This device traps plasma in a self-organized quasi-stable structure; where the particle motion makes an internal magnetic field which then traps itself.<ref name="Freidberg2007">{{cite book|first=Jeffrey P. |last=Freidberg|title=Plasma Physics and Fusion Energy|url={{google books |plainurl=y |id=ZGU-ngEACAAJ}}|date= 2007|publisher=Cambridge University Press|isbn=978-0521851077}}</ref> |
|||
* [[Spheromak]]: Similar to a field-reversed configuration, a semi-stable plasma structure made by using the plasmas' self-generated magnetic field. A spheromak has both toroidal and poloidal fields, while a field-reversed configuration has no toroidal field.<ref>{{cite book |title=Magnetic Fusion Technology |publisher=Springer London |year=2013 |isbn=978-1447155553 |editor-last=Dolan |editor-first=Thomas J. |series=Lecture Notes in Energy |volume=19 |location=London, England |pages=30–40 |language=en |doi=10.1007/978-1-4471-5556-0 |issn=2195-1284 }}</ref> |
|||
* [[Dynomak]] is a spheromak that is formed and sustained using continuous [[magnetic flux]] injection.<ref>D. A. Sutherland, T. R. Jarboe et al., "The dynomak: An advanced spheromak reactor concept with imposed-dynamo current drive and next-generation nuclear power technologies", Fusion Engineering and Design, Volume 89, Issue 4, April 2014, pp. 412–425.</ref><ref>Jarboe, T. R., et al. "Spheromak formation by steady inductive helicity injection." Physical Review Letters 97.11 (2006): 115003</ref><ref>Jarboe, T. R., et al. "Recent results from the HIT-SI experiment." Nuclear Fusion 51.6 (2011): 063029</ref> |
|||
* [[Reversed field pinch]]: Here the plasma moves inside a ring. It has an internal magnetic field. Moving out from the center of this ring, the magnetic field reverses direction. |
|||
=== Inertial confinement === |
|||
{{Main|Inertial confinement fusion}} |
|||
[[File:NIF output over 11 years without legend.png|upright=1.7|thumb|alt=Plot of NIF results from 2012 to 2022|Plot of NIF results from 2012 to 2022]] |
|||
* Indirect drive: Lasers heat a structure known as a [[Hohlraum]] that becomes so hot it begins to radiate [[x-ray]] light. These x-rays heat a fuel pellet, causing it to collapse inward to compress the fuel. The largest system using this method is the [[National Ignition Facility]], followed closely by [[Laser Mégajoule]].<ref name="Nuckolls, John 1972">{{cite journal | last1 = Nuckolls | first1 = John | last2 = Wood | first2 = Lowell | last3 = Thiessen | first3 = Albert | last4 = Zimmerman | first4 = George | s2cid = 45684425 | year = 1972 | title = Laser Compression of Matter to Super-High Densities: Thermonuclear (CTR) Applications | journal = Nature | volume = 239 | issue = 5368| pages = 139–142 | doi = 10.1038/239139a0 |bibcode = 1972Natur.239..139N }}</ref> |
|||
* Direct drive: Lasers directly heat the fuel pellet. Notable direct drive experiments have been conducted at the [[Laboratory for Laser Energetics]] (LLE) and the [[GEKKO XII]] facilities. Good implosions require fuel pellets with close to a perfect shape in order to generate a symmetrical inward [[shock wave]] that produces the high-density plasma.{{citation needed|date=August 2023}} |
|||
* Fast ignition: This method uses two laser blasts. The first blast compresses the fusion fuel, while the second ignites it. {{as of|2019}} this technique had lost favor for energy production.<ref>{{Cite book|last=Turrell|first=Arthur |title=How to Build a Star: the science of nuclear fusion and the quest to harness its power|date=2021|publisher=Weidenfeld & Nicolson |isbn=978-1474611596|location=Place of publication not identified|language=en|oclc=1048447399}}</ref> |
|||
* [[Magneto-inertial fusion]] or [[Magnetized Liner Inertial Fusion]]: This combines a laser pulse with a magnetic pinch. The pinch community refers to it as magnetized liner inertial fusion while the ICF community refers to it as magneto-inertial fusion.<ref name="IFSA2007">{{cite journal |last=Thio |first=Y. C. F. |date=1 April 2008 |title=Status of the US program in magneto-inertial fusion |journal=Journal of Physics: Conference Series |publisher=IOP Publishing |volume=112 |issue=4 |page=042084 |bibcode=2008JPhCS.112d2084T |doi=10.1088/1742-6596/112/4/042084 |issn=1742-6596 |doi-access=free}}</ref> |
|||
* Ion Beams: Ion beams replace laser beams to heat the fuel.<ref>{{cite conference |last1=Sharp |first1=W. M. |last2=Friedman |first2=A. |display-authors=1 |year=2011 |title=Inertial Fusion Driven by Intense Heavy-Ion Beams |url=https://accelconf.web.cern.ch/AccelConf/PAC2011/papers/weoas1.pdf |conference=Proceedings of 2011 Particle Accelerator Conference |location=New York, New York, USA |page=1386 |archive-url=https://web.archive.org/web/20171126054145/http://accelconf.web.cern.ch/AccelConf/PAC2011/papers/weoas1.pdf |archive-date=November 26, 2017 |access-date=August 3, 2019 |url-status=dead}}</ref> The main difference is that the beam has momentum due to mass, whereas lasers do not. As of 2019 it appears unlikely that ion beams can be sufficiently focused spatially and in time. |
|||
* [[Z Pulsed Power Facility|Z-machine]]: Sends an electric current through thin tungsten wires, heating them sufficiently to generate x-rays. Like the indirect drive approach, these x-rays then compress a fuel capsule. |
|||
=== Magnetic or electric pinches === |
|||
{{Main|Pinch (plasma physics)}} |
|||
* ''[[Z-pinch]]:'' A current travels in the z-direction through the plasma. The current generates a magnetic field that compresses the plasma. Pinches were the first method for human-made controlled fusion.<ref name="Seife, Charles 2008">{{cite book |last=Seife |first=Charles |title=Sun in a bottle: the strange history of fusion and the science of wishful thinking |publisher=Viking |year=2008 |isbn=978-0670020331 |location=New York |language=en-us |oclc=213765956}}</ref><ref name="Phillips, James 2013">{{cite journal|last=Phillips|first=James|title=Magnetic Fusion|journal=Los Alamos Science|date=1983|pages=64–67|access-date=4 Apr 2013|url=https://permalink.lanl.gov/object/tr?what=info:lanl-repo/lareport/LA-UR-83-5080|archive-url=https://web.archive.org/web/20161223163131/http://permalink.lanl.gov/object/tr?what=info%3Alanl-repo%2Flareport%2FLA-UR-83-5080|archive-date=December 23, 2016|url-status=dead}}</ref> The z-pinch has inherent instabilities that limit its compression and heating to values too low for practical fusion. The largest such machine, the UK's [[ZETA (fusion reactor)|ZETA]], was the last major experiment of the sort. The problems in z-pinch led to the tokamak design. The [[dense plasma focus]] is a possibly superior variation. |
|||
* ''[[Theta-pinch]]:'' A current circles around the outside of a plasma column, in the theta direction. This induces a magnetic field running down the center of the plasma, as opposed to around it. The early theta-pinch device Scylla was the first to conclusively demonstrate fusion, but later work demonstrated it had inherent limits that made it uninteresting for power production. |
|||
* ''Sheared Flow Stabilized Z-Pinch:'' Research at the [[University of Washington]] under Uri Shumlak investigated the use of sheared-flow stabilization to smooth out the instabilities of Z-pinch reactors. This involves accelerating neutral gas along the axis of the pinch. Experimental machines included the FuZE and Zap Flow Z-Pinch experimental reactors.<ref>{{Cite web|date=2014-11-07|title=Flow Z-Pinch Experiments|url=https://www.aa.washington.edu/research/ZaP|access-date=2020-10-11|website=Aeronautics and Astronautics|language=en}}</ref> In 2017, British technology investor and entrepreneur [[Benj Conway]], together with physicists Brian Nelson and Uri Shumlak, co-founded Zap Energy to attempt to commercialize the technology for power production.<ref>{{cite web |url=https://www.zapenergyinc.com/ |publisher=Zap Energy |title=Zap Energy |access-date=February 13, 2020 |archive-url=https://web.archive.org/web/20200213065735/https://www.zapenergyinc.com/ |archive-date=February 13, 2020 |url-status=dead }}</ref><ref>{{Cite web|title=Board of Directors|url=https://www.zapenergyinc.com/board|access-date=2020-09-08|website=ZAP ENERGY|language=en-US}}</ref><ref>{{Cite web|date=2020-08-13|title=Chevron announces investment in nuclear fusion start-up Zap Energy|url=https://www.power-technology.com/news/chevron-announces-investment-nuclear-fusion-start-up-company-zap-energy/|access-date=2020-09-08|website=Power Technology {{!}} Energy News and Market Analysis|language=en-GB}}</ref> |
|||
* ''Screw Pinch:'' This method combines a theta and z-pinch for improved stabilization.<ref>{{cite journal | last1=Srivastava | first1=Krishna M. | last2=Vyas | first2=D. N. | title=Non-linear analysis of the stability of the Screw Pinch | journal=Astrophysics and Space Science | publisher=Springer Nature | volume=86 | issue=1 | year=1982 | issn=0004-640X | doi=10.1007/bf00651831 | pages=71–89| bibcode=1982Ap&SS..86...71S | s2cid=121575638 }}</ref> |
|||
=== Inertial electrostatic confinement === |
|||
{{Main|Inertial electrostatic confinement}} |
|||
* ''[[Fusor]]:'' An electric field heats ions to fusion conditions. The machine typically uses two spherical cages, a cathode inside the anode, inside a vacuum. These machines are not considered a viable approach to net power because of their high [[Electrical conductor|conduction]] and [[radiation]] losses.<ref>{{cite journal | last=Rider | first=Todd H. | title=A general critique of inertial-electrostatic confinement fusion systems | journal=Physics of Plasmas | publisher=AIP Publishing | volume=2 | issue=6 | year=1995 | issn=1070-664X | doi=10.1063/1.871273 | pages=1853–1872| bibcode=1995PhPl....2.1853R | hdl=1721.1/29869 | s2cid=12336904 | hdl-access=free }}</ref> They are simple enough to build that amateurs have fused atoms using them.<ref>{{cite web | last=Clynes | first=Tom | title=The Boy Who Played With Fusion | website=Popular Science | date=14 February 2012 | url=https://www.popsci.com/science/article/2012-02/boy-who-played-fusion/ | access-date=3 August 2019}}</ref> |
|||
* ''[[Polywell]]:'' Attempts to combine magnetic confinement with electrostatic fields, to avoid the [[Electrical conductor|conduction]] losses generated by the cage.<ref>US patent 5,160,695, Robert W. Bussard, "Method and apparatus for creating and controlling nuclear fusion reactions", issued 1992-11-03</ref> |
|||
=== Other === |
|||
* ''[[Magnetized target fusion]]:'' Confines hot plasma using a magnetic field and squeezes it using inertia. Examples include [[LANL]] FRX-L machine,<ref>{{Cite journal|last1=Taccetti|first1=J. M.|last2=Intrator|first2=T. P.|last3=Wurden|first3=G. A.|last4=Zhang|first4=S. Y.|last5=Aragonez|first5=R.|last6=Assmus|first6=P. N.|last7=Bass|first7=C. M.|last8=Carey|first8=C.|last9=deVries|first9=S. A.|last10=Fienup|first10=W. J.|last11=Furno|first11=I.|date=2003-09-25|title=FRX-L: A field-reversed configuration plasma injector for magnetized target fusion|url=https://aip.scitation.org/doi/10.1063/1.1606534|journal=Review of Scientific Instruments|volume=74|issue=10|pages=4314–4323|doi=10.1063/1.1606534|bibcode=2003RScI...74.4314T|issn=0034-6748}}</ref> [[General Fusion]] (piston compression with liquid metal liner), HyperJet Fusion (plasma jet compression with plasma liner).<ref>{{Cite journal|last1=Hsu|first1=S. C.|last2=Awe|first2=T. J.|last3=Brockington|first3=S.|last4=Case|first4=A.|last5=Cassibry|first5=J. T.|last6=Kagan|first6=G.|last7=Messer|first7=S. J.|last8=Stanic|first8=M.|last9=Tang|first9=X.|last10=Welch|first10=D. R.|last11=Witherspoon|first11=F. D.|date=2012|title=Spherically Imploding Plasma Liners as a Standoff Driver for Magnetoinertial Fusion|url=https://ieeexplore.ieee.org/document/6168279/?denied=|journal=IEEE Transactions on Plasma Science|volume=40|issue=5|pages=1287–1298|doi=10.1109/TPS.2012.2186829|bibcode=2012ITPS...40.1287H|s2cid=32998378|issn=1939-9375}}</ref><ref name=5big/> |
|||
* ''Uncontrolled:'' Fusion has been initiated by man, using uncontrolled fission explosions to stimulate fusion. Early proposals for fusion power included using bombs to initiate reactions. See [[Project PACER]]. |
|||
* ''Beam fusion:'' A beam of high energy particles fired at another beam or target can initiate fusion. This was used in the 1970s and 1980s to study the cross sections of fusion reactions.<ref name="osti.gov" /> However beam systems cannot be used for power because keeping a beam coherent takes more energy than comes from fusion. |
|||
* ''[[Muon-catalyzed fusion]]:'' This approach replaces [[electron]]s in [[diatomic molecule]]s of [[isotope]]s of [[hydrogen]] with [[muon]]s—more massive particles with the same [[electric charge]]. Their greater mass compresses the nuclei enough such that the [[strong interaction]] can cause fusion.{{sfn|Nagamine|2003}} As of 2007 producing muons required more energy than can be obtained from muon-catalyzed fusion.<ref>{{Cite book |last=Nagamine |first=K. |title=Introductory muon science |date=2007 |publisher=Cambridge University Press |isbn=978-0521038201 |location=Cambridge, England |language=en |oclc=124025585}}</ref> |
|||
* ''[[Lattice confinement fusion]]:'' Lattice confinement fusion ('''LCF''') is a type of [[nuclear fusion]] in which [[deuteron]]-saturated metals are exposed to [[gamma radiation]] or ion beams, such as in an [[Inertial electrostatic confinement|IEC fusor]], avoiding the confined high-temperature plasmas used in other methods of fusion.<ref>{{Cite web|url=https://spectrum.ieee.org/lattice-confinement-fusion|title=NASA's New Shortcut to Fusion Power|last1=Baramsai |first1=Bayardadrakh |last2=Benyo |first2=Theresa |last3=Forsley |first3=Lawrence |last4=Steinetz |first4=Bruce |date=February 27, 2022|website=IEEE Spectrum}}</ref><ref name="erbium">{{Cite journal|url=http://dx.doi.org/10.1103/PhysRevC.101.044610|title=Novel nuclear reactions observed in bremsstrahlung-irradiated deuterated metals|first1=Bruce M.|last1=Steinetz|first2=Theresa L.|last2=Benyo|first3=Arnon|last3=Chait|first4=Robert C.|last4=Hendricks|first5=Lawrence P.|last5=Forsley|first6=Bayarbadrakh|last6=Baramsai|first7=Philip B.|last7=Ugorowski|first8=Michael D.|last8=Becks|first9=Vladimir|last9=Pines|first10=Marianna|last10=Pines|first11=Richard E.|last11=Martin|first12=Nicholas|last12=Penney|first13=Gustave C.|last13=Fralick|first14=Carl E.|last14=Sandifer|date=April 20, 2020|journal=Physical Review C|volume=101|issue=4|pages=044610|via=APS|doi=10.1103/physrevc.101.044610|bibcode=2020PhRvC.101d4610S|s2cid=219083603}}</ref> |
|||
== Common tools == |
|||
Many approaches, equipment, and mechanisms are employed across multiple projects to address fusion heating, measurement, and power production.<ref>{{Cite journal|date=1972|title=Plasma Physics|journal=Government Reports Announcements|volume=72|pages=194}}</ref> |
|||
=== Neural networks === |
|||
A deep reinforcement learning system has been used to control a [[tokamak]]-based reactor. The AI was able to manipulate the magnetic coils to manage the plasma. The system was able to continuously adjust to maintain appropriate behavior (more complex than step-based systems). In 2014, Google began working with California-based fusion company [[TAE Technologies]] to control the [[Joint European Torus]] (JET) to predict plasma behavior.<ref>{{Cite magazine|last=Katwala|first=Amit|date=February 16, 2022|title=DeepMind Has Trained an AI to Control Nuclear Fusion|language=en-US|magazine=Wired|url=https://www.wired.com/story/deepmind-ai-nuclear-fusion/|access-date=2022-02-17|issn=1059-1028}}</ref> [[DeepMind]] has also developed a control scheme with [[Tokamak à configuration variable|TCV]].<ref>{{cite magazine | url=https://www.wired.com/story/deepmind-ai-nuclear-fusion/ | title=DeepMind Has Trained an AI to Control Nuclear Fusion | magazine=Wired | last1=Katwala | first1=Amit }}</ref> |
|||
=== Heating === |
|||
{{Main|Dielectric heating|Magnetic reconnection|Inertial electrostatic confinement|Neutral beam injection}} |
|||
* Electrostatic heating: an electric field can do [[work (thermodynamics)|work]] on charged ions or electrons, heating them.<ref>{{Cite book|last=Miley, George H. |title=Inertial electrostatic confinement (IEC) fusion : fundamentals and applications|date=2013|publisher=Springer|others=Murali, S. Krupakar|isbn=978-1461493389|location=Dordrecht|oclc=878605320}}</ref> |
|||
* [[Neutral-beam injection|Neutral beam injection]]: hydrogen is ionized and accelerated by an electric field to form a charged beam that is shone through a source of neutral hydrogen gas towards the plasma which itself is ionized and contained by a magnetic field. Some of the intermediate hydrogen gas is accelerated towards the plasma by collisions with the charged beam while remaining neutral: this neutral beam is thus unaffected by the magnetic field and so reaches the plasma. Once inside the plasma the neutral beam transmits energy to the plasma by collisions which ionize it and allow it to be contained by the magnetic field, thereby both heating and refueling the reactor in one operation. The remainder of the charged beam is diverted by magnetic fields onto cooled beam dumps.<ref>{{Cite book |last=Kunkel |first=W. B. |title=Fusion |publisher=Lawrence Livermore National Laboratory |year=1981 |isbn=978-0126852417 |editor-last=Teller |editor-first=E. |chapter=Neutral-beam injection}}</ref> |
|||
* Radio frequency heating: a radio wave causes the plasma to oscillate (i.e., [[microwave oven]]). This is also known as [[electron cyclotron resonance heating]], using for example [[gyrotron]]s, or [[dielectric heating]].<ref>{{Cite journal|last1=Erckmann|first1=V|last2=Gasparino|first2=U|date=1994-12-01|title=Electron cyclotron resonance heating and current drive in toroidal fusion plasmas|url=https://iopscience.iop.org/article/10.1088/0741-3335/36/12/001|journal=Plasma Physics and Controlled Fusion|volume=36|issue=12|pages=1869–1962|bibcode=1994PPCF...36.1869E|doi=10.1088/0741-3335/36/12/001|s2cid=250897078|issn=0741-3335}}</ref> |
|||
* [[Magnetic reconnection]]: when plasma gets dense, its electromagnetic properties can change, which can lead to [[magnetic reconnection]]. Reconnection helps fusion because it instantly dumps energy into a plasma, heating it quickly. Up to 45% of the magnetic field energy can heat the ions.<ref>{{Cite journal|last1=Ono|first1=Y.|last2=Tanabe|first2=H.|last3=Yamada|first3=T.|last4=Gi|first4=K.|last5=Watanabe|first5=T.|last6=Ii|first6=T.|last7=Gryaznevich|first7=M.|last8=Scannell|first8=R.|last9=Conway|first9=N.|last10=Crowley|first10=B.|last11=Michael|first11=C.|date=2015-05-01|title=High power heating of magnetic reconnection in merging tokamak experiments|url=https://aip.scitation.org/doi/10.1063/1.4920944|journal=Physics of Plasmas|volume=22|issue=5|pages=055708|bibcode=2015PhPl...22e5708O|doi=10.1063/1.4920944|issn=1070-664X|hdl=1885/28549|hdl-access=free}}</ref><ref>{{Cite journal|last1=Yamada|first1=M.|last2=Chen|first2=L.-J.|last3=Yoo|first3=J.|last4=Wang|first4=S.|last5=Fox|first5=W.|last6=Jara-Almonte|first6=J.|last7=Ji|first7=H.|last8=Daughton|first8=W.|last9=Le|first9=A.|last10=Burch|first10=J.|last11=Giles|first11=B.|date=2018-12-06|title=The two-fluid dynamics and energetics of the asymmetric magnetic reconnection in laboratory and space plasmas|url= |journal=Nature Communications|language=en|volume=9|issue=1|pages=5223|bibcode=2018NatCo...9.5223Y|doi=10.1038/s41467-018-07680-2|issn=2041-1723|pmc=6283883|pmid=30523290}}</ref> |
|||
* Magnetic oscillations: varying electric currents can be supplied to magnetic coils that heat plasma confined within a magnetic wall.<ref>McGuire, Thomas. Heating Plasma for Fusion Power Using Magnetic Field Oscillations. Baker Botts LLP, assignee. Issued: 4/2/14, Patent 14/243,447. N.d. Print.</ref> |
|||
* Antiproton annihilation: [[antiproton]]s injected into a mass of fusion fuel can induce thermonuclear reactions. This possibility as a method of spacecraft propulsion, known as [[antimatter-catalyzed nuclear pulse propulsion]], was investigated at [[Pennsylvania State University]] in connection with the proposed [[AIMStar]] project.{{Citation needed|date=June 2021}} |
|||
=== Measurement === |
|||
{{Main|Flux loop|Langmuir probe|Neutron detection|Thomson scattering|X-ray detector}} |
|||
The diagnostics of a fusion scientific reactor are extremely complex and varied.<ref>{{Citation|title=Towards a fusion reactor|url=http://dx.doi.org/10.1887/0750307056/b888c9|work=Nuclear Fusion|year=2002|publisher=IOP Publishing Ltd|doi=10.1887/0750307056/b888c9|isbn=0750307056|access-date=2021-12-12 }}</ref> The diagnostics required for a fusion power reactor will be various but less complicated than those of a scientific reactor as by the time of commercialization, many real-time feedback and control diagnostics will have been perfected. However, the operating environment of a commercial fusion reactor will be harsher for diagnostic systems than in a scientific reactor because continuous operations may involve higher plasma temperatures and higher levels of neutron irradiation. In many proposed approaches, commercialization will require the additional ability to measure and separate diverter gases, for example helium and impurities, and to monitor fuel breeding, for instance the state of a tritium breeding liquid lithium liner.<ref>{{Citation|last1=Pearson|first1=Richard J|title=Review of approaches to fusion energy|date=2020|url=http://dx.doi.org/10.1088/978-0-7503-2719-0ch2|work=Commercialising Fusion Energy|publisher=IOP Publishing|access-date=2021-12-12|last2=Takeda|first2=Shutaro|doi=10.1088/978-0-7503-2719-0ch2|isbn=978-0750327190|s2cid=234561187}}</ref> The following are some basic techniques. |
|||
* [[Flux loop]]: a loop of wire is inserted into the magnetic field. As the field passes through the loop, a current is made. The current measures the total magnetic flux through that loop. This has been used on the [[National Compact Stellarator Experiment]],<ref>{{Cite book|last1=Labik|first1=George|last2=Brown|first2=Tom|last3=Johnson|first3=Dave|last4=Pomphrey|first4=Neil|last5=Stratton|first5=Brentley|last6=Viola|first6=Michael|last7=Zarnstorff|first7=Michael|last8=Duco|first8=Mike|last9=Edwards|first9=John|last10=Cole|first10=Mike|last11=Lazarus|first11=Ed|title=2007 IEEE 22nd Symposium on Fusion Engineering |chapter=National Compact Stellarator Experiment Vacuum Vessel External Flux Loops Design and Installation |date=2007|chapter-url=https://ieeexplore.ieee.org/document/4337935|pages=1–3|doi=10.1109/FUSION.2007.4337935|isbn=978-1424411931|s2cid=9298179}}</ref> the [[polywell]],<ref>{{Cite journal|last1=Park|first1=Jaeyoung|last2=Krall|first2=Nicholas A.|last3=Sieck|first3=Paul E.|last4=Offermann|first4=Dustin T.|last5=Skillicorn|first5=Michael|last6=Sanchez|first6=Andrew|last7=Davis|first7=Kevin|last8=Alderson|first8=Eric|last9=Lapenta|first9=Giovanni|date=2014-06-01|title=High Energy Electron Confinement in a Magnetic Cusp Configuration|journal=Physical Review X|volume=5|issue=2|pages=021024|arxiv=1406.0133|bibcode=2015PhRvX...5b1024P|doi=10.1103/PhysRevX.5.021024|s2cid=118478508}}</ref> and the [[Levitated dipole|LDX]] machines. A [[Langmuir probe]], a metal object placed in a plasma, can be employed. A potential is applied to it, giving it a [[voltage]] against the surrounding plasma. The metal collects charged particles, drawing a current. As the voltage changes, the current changes. This makes an [[Current–voltage characteristic|IV Curve]]. The IV-curve can be used to determine the local plasma density, potential and temperature.<ref>{{cite journal|last1=Mott-Smith|first1=H. M.|last2=Langmuir|first2=Irving|date=1 September 1926|title=The Theory of Collectors in Gaseous Discharges|journal=Physical Review|publisher=American Physical Society (APS)|volume=28|issue=4|pages=727–763|bibcode=1926PhRv...28..727M|doi=10.1103/physrev.28.727|issn=0031-899X}}</ref> |
|||
* [[Thomson scattering]]: "Light scatters" from plasma can be used to reconstruct plasma behavior, including density and temperature. It is common in [[Inertial confinement fusion]],<ref>{{cite journal | last1=Esarey | first1=Eric | last2=Ride | first2=Sally K. | last3=Sprangle | first3=Phillip | title=Nonlinear Thomson scattering of intense laser pulses from beams and plasmas | journal=Physical Review E | publisher=American Physical Society (APS) | volume=48 | issue=4 | date=1 September 1993 | issn=1063-651X | doi=10.1103/physreve.48.3003 | pmid=9960936 | pages=3003–3021| bibcode=1993PhRvE..48.3003E }}</ref> [[Tokamak]]s,<ref>{{Cite journal |last1=Kantor |first1=M. Yu |last2=Donné |first2=A. J. H. |last3=Jaspers |first3=R. |last4=van der Meiden |first4=H. J. |date=2009-02-26 |title=Thomson scattering system on the TEXTOR tokamak using a multi-pass laser beam configuration |url=https://iopscience.iop.org/article/10.1088/0741-3335/51/5/055002 |journal=Plasma Physics and Controlled Fusion |language=en |volume=51 |issue=5 |pages=055002 |bibcode=2009PPCF...51e5002K |doi=10.1088/0741-3335/51/5/055002 |issn=0741-3335 |s2cid=123495440}}</ref> and [[fusor]]s. In ICF systems, firing a second beam into a gold foil adjacent to the target makes x-rays that traverse the plasma. In tokamaks, this can be done using mirrors and detectors to reflect light. |
|||
* [[Neutron detection|Neutron detectors]]: [[Neutron detection#Types of neutron detectors|Several types of neutron detectors]] can record the rate at which neutrons are produced.<ref>{{Cite book|last=Tsoulfanidis|first=Nicholas|url=http://archive.org/details/measurementdetec00tsou|title=Measurement and detection of radiation|date=1995|publisher=Washington, DC : Taylor & Francis|others=Library Genesis|isbn=978-1560323174}}</ref><ref>{{Cite book|last=Knoll, Glenn F. |title=Radiation detection and measurement|date=2010|publisher=John Wiley|isbn=978-0470131480|edition=4th|location=Hoboken, NJ|oclc=612350364}}</ref> |
|||
* [[X-ray detectors]] Visible, IR, UV, and X-rays are emitted anytime a particle changes velocity.<ref>{{Cite journal|last=Larmor|first=Joseph|date=1897-01-01|title=IX. A dynamical theory of the electric and luminiferous medium. Part III. relations with material media|journal=Philosophical Transactions of the Royal Society of London. Series A, Containing Papers of a Mathematical or Physical Character|volume=190|pages=205–300|doi=10.1098/rsta.1897.0020|bibcode=1897RSPTA.190..205L|doi-access=free}}</ref> If the reason is deflection by a magnetic field, the radiation is [[cyclotron]] radiation at low speeds and [[synchrotron]] radiation at high speeds. If the reason is deflection by another particle, plasma radiates X-rays, known as [[Bremsstrahlung]] radiation.<ref>{{Cite book |title=Diagnostics for experimental thermonuclear fusion reactors 2|date=1998|publisher=Springer |veditors=Stott PE, Gorini G, Prandoni P, Sindoni E |isbn=978-1461553533|location=New York|oclc=828735433}}</ref> |
|||
=== Power production === |
|||
Neutron blankets absorb neutrons, which heats the blanket. Power can be extracted from the blanket in various ways: |
|||
* [[Steam turbine]]s can be driven by heat transferred into a [[working fluid]] that turns into steam, driving electric generators.<ref>{{Cite journal|last1=Ishiyama|first1=Shintaro|last2=Muto|first2=Yasushi|last3=Kato|first3=Yasuyoshi|last4=Nishio|first4=Satoshi|last5=Hayashi|first5=Takumi|last6=Nomoto|first6=Yasunobu|date=2008-03-01|title=Study of steam, helium and supercritical CO2 turbine power generations in prototype fusion power reactor|url=http://www.sciencedirect.com/science/article/pii/S0149197007001552|journal=Progress in Nuclear Energy|series=Innovative Nuclear Energy Systems for Sustainable Development of the World. Proceedings of the Second COE-INES International Symposium, INES-2, November 26–30, 2006, Yokohama, Japan|language=en|volume=50|issue=2|pages=325–332|doi=10.1016/j.pnucene.2007.11.078|issn=0149-1970}}</ref> |
|||
* Neutron blankets: These neutrons can regenerate spent fission fuel.<ref>{{cite web |last1=Anklam |first1=T. |last2=Simon |first2=A. J. |last3=Powers |first3=S. |last4=Meier |first4=W. R. |date=December 2, 2010 |title=LIFE: The Case for Early Commercialization of Fusion Energy |url=https://e-reports-ext.llnl.gov/pdf/459730.pdf |url-status=dead |archive-url=https://web.archive.org/web/20150904055903/https://e-reports-ext.llnl.gov/pdf/459730.pdf |archive-date=4 September 2015 |access-date=30 October 2014 |publisher=Lawrence Livermore National Laboratory, LLNL-JRNL-463536}}</ref> Tritium can be produced using a breeder blanket of liquid lithium or a helium cooled pebble bed made of lithium-bearing ceramic pebbles.<ref name="l2s">{{cite journal |last1=Hanaor |first1=D. A. H. |last2=Kolb |first2=M. H. H. |last3=Gan |first3=Y. |last4=Kamlah |first4=M. |last5=Knitter |first5=R. |year=2014 |title=Solution based synthesis of mixed-phase materials in the Li<sub>2</sub>TiO<sub>3</sub>-Li<sub>4</sub>SiO<sub>4</sub> system |journal=Journal of Nuclear Materials |volume=456 |pages=151–161 |arxiv=1410.7128 |bibcode=2015JNuM..456..151H |doi=10.1016/j.jnucmat.2014.09.028 |s2cid=94426898}}</ref> |
|||
* [[Direct energy conversion|Direct conversion]]: The [[kinetic energy]] of a particle can be converted into [[voltage]].<ref name="Post 99–111" /> It was first suggested by [[Richard F. Post]] in conjunction with [[magnetic mirror]]s, in the late 1960s. It has been proposed for [[Field-Reversed Configuration]]s as well as [[Dense Plasma Focus]] devices. The process converts a large fraction of the random energy of the fusion products into directed motion. The particles are then collected on electrodes at various large electrical potentials. This method has demonstrated an experimental efficiency of 48 percent.<ref>{{Cite journal|last1=Barr|first1=William L.|last2=Moir|first2=Ralph W.|date=1983-01-01|title=Test Results on Plasma Direct Converters|url=https://doi.org/10.13182/FST83-A20820|journal=Nuclear Technology – Fusion|volume=3|issue=1|pages=98–111|doi=10.13182/FST83-A20820|bibcode=1983NucTF...3...98B |issn=0272-3921}}</ref> |
|||
* [[Traveling-wave tube]]s pass charged helium atoms at several megavolts and just coming off the fusion reaction through a tube with a coil of wire around the outside. This passing charge at high voltage pulls electricity through the wire. |
|||
=== Confinement === |
|||
[[File:IFE and MFE parameter space.svg|thumb|upright=1.75|Parameter space occupied by [[inertial fusion energy]] and [[magnetic fusion energy]] devices as of the mid-1990s. The regime allowing thermonuclear ignition with high gain lies near the upper right corner of the plot.]] |
|||
Confinement refers to all the conditions necessary to keep a plasma dense and hot long enough to undergo fusion. General principles: |
|||
* [[Mechanical equilibrium|Equilibrium]]: The forces acting on the plasma must be balanced. One exception is [[inertial confinement fusion|inertial confinement]], where the fusion must occur faster than the dispersal time. |
|||
* [[Plasma stability|Stability]]: The plasma must be constructed so that disturbances will not lead to the plasma dispersing. |
|||
* Transport or [[conduction (heat)|conduction]]: The loss of material must be sufficiently slow.<ref name="Lawson"/> The plasma carries energy off with it, so rapid loss of material will disrupt fusion. Material can be lost by transport into different regions or [[conduction (heat)|conduction]] through a solid or liquid. |
|||
To produce self-sustaining fusion, part of the energy released by the reaction must be used to heat new reactants and maintain the conditions for fusion. |
|||
==== Magnetic confinement ==== |
|||
===== Magnetic Mirror ===== |
|||
[[Magnetic mirror]] effect. If a particle follows the field line and enters a region of higher field strength, the particles can be reflected. Several devices apply this effect. The most famous was the magnetic mirror machines, a series of devices built at LLNL from the 1960s to the 1980s.<ref name=Booth>{{cite journal|last=Booth|first=William|title=Fusion's $372-Million Mothball|journal=Science|date=9 Oct 1987|volume=238|issue=4824|pages=152–155|doi= 10.1126/science.238.4824.152|pmid=17800453|bibcode=1987Sci...238..152B}}</ref> Other examples include magnetic bottles and [[Biconic cusp]].<ref>{{Cite book|last=Grad|first=Harold |title=Containment in cusped plasma systems (classic reprint).|date=2016|publisher=Forgotten Books |isbn=978-1333477035|location=<!-- Place of publication not identified -->|language=en|oclc=980257709}}</ref> Because the mirror machines were straight, they had some advantages over ring-shaped designs. The mirrors were easier to construct and maintain and [[Direct energy conversion|direct conversion]] energy capture was easier to implement.<ref name="ReferenceA"/> Poor confinement has led this approach to be abandoned, except in the polywell design.<ref>{{Cite web|last=Lee|first=Chris|date=2015-06-22|title=Magnetic mirror holds promise for fusion|url=https://arstechnica.com/science/2015/06/magnetic-mirror-holds-promise-for-fusion/|access-date=2020-10-11|website=Ars Technica|language=en-us}}</ref> |
|||
===== Magnetic loops ===== |
|||
Magnetic loops bend the field lines back on themselves, either in circles or more commonly in nested [[torus|toroidal]] surfaces. The most highly developed systems of this type are the [[tokamak]], the stellarator, and the reversed field pinch. [[Compact toroid]]s, especially the field-reversed configuration and the spheromak, attempt to combine the advantages of toroidal magnetic surfaces with those of a [[simply connected space|simply connected]] (non-toroidal) machine, resulting in a mechanically simpler and smaller confinement area. |
|||
==== Inertial confinement ==== |
|||
[[File:Electra Laser Generates 90K Shots.webm|thumb|alt=The Electra Laser at Naval Research Laboratory demonstrates 90,000 shots in 10 hours, repetition needed for IFE power plant.|The Electra Laser at Naval Research Laboratory demonstrates 90,000 shots in 10 hours, repetition needed for IFE power plant.]] |
|||
Inertial confinement is the use of rapid implosion to heat and confine plasma. A shell surrounding the fuel is imploded using a direct laser blast (direct drive), a secondary x-ray blast (indirect drive), or heavy beams. The fuel must be compressed to about 30 times solid density with energetic beams. Direct drive can in principle be efficient, but insufficient uniformity has prevented success.<ref name="confinement">{{Cite book|last=Pfalzner, Susanne |title=An introduction to inertial confinement fusion|date=2006|publisher=Taylor & Francis/CRC Press|isbn=1420011847|location=New York|oclc=72564680}}</ref><sup>:19–20</sup> Indirect drive uses beams to heat a shell, driving the shell to radiate [[x-rays]], which then implode the pellet. The beams are commonly laser beams, but ion and electron beams have been investigated.<ref name="confinement" /><sup>:182–193</sup> |
|||
===== Electrostatic confinement ===== |
|||
[[Inertial electrostatic confinement|Electrostatic confinement fusion]] devices use electrostatic fields. The best known is the [[fusor]]. This device has a cathode inside an anode wire cage. Positive ions fly towards the negative inner cage, and are heated by the electric field in the process. If they miss the inner cage they can collide and fuse. Ions typically hit the cathode, however, creating prohibitory high [[conduction (heat)|conduction]] losses. Fusion rates in [[fusor]]s are low because of competing physical effects, such as energy loss in the form of light radiation.<ref name="Thorson1996">{{cite book|first=Timothy A. |last=Thorson|title=Ion flow and fusion reactivity characterization of a spherically convergent ion focus|url={{google books |plainurl=y |id=k6zVAAAAMAAJ}}|year=1996|publisher=University of Wisconsin, Madison}}</ref> Designs have been proposed to avoid the problems associated with the cage, by generating the field using a non-neutral cloud. These include a plasma oscillating device,<ref>{{Cite journal|last1=Barnes|first1=D. C.|last2=Nebel|first2=R. A.|date=July 1998|title=Stable, thermal equilibrium, large-amplitude, spherical plasma oscillations in electrostatic confinement devices|url=http://dx.doi.org/10.1063/1.872933|journal=Physics of Plasmas|volume=5|issue=7|pages=2498–2503|doi=10.1063/1.872933|bibcode=1998PhPl....5.2498B|issn=1070-664X}}</ref> a magnetically shielded-grid,<ref>{{Cite journal|last1=Hedditch|first1=John|last2=Bowden-Reid|first2=Richard|last3=Khachan|first3=Joe|date=October 2015|title=Fusion in a magnetically-shielded-grid inertial electrostatic confinement device|journal=Physics of Plasmas|volume=22|issue=10|pages=102705|doi=10.1063/1.4933213|issn=1070-664X|arxiv=1510.01788|bibcode=2015PhPl...22j2705H }}</ref> a [[penning trap]], the [[polywell]],<ref>{{cite journal | last1 = Carr | first1 = M. | last2 = Khachan | first2 = J. | year = 2013 | title = A biased probe analysis of potential well formation in an electron only, low beta Polywell magnetic field | url = https://zenodo.org/record/1244056| journal = Physics of Plasmas | volume = 20 | issue = 5| page = 052504 | doi = 10.1063/1.4804279 | bibcode = 2013PhPl...20e2504C }}</ref> and the F1 cathode driver concept.<ref>{{Cite book|last1=Sieckand|first1=Paul|url=https://arpa-e.energy.gov/sites/default/files/3_VOLBERG.pdf|title=Fusion One Corporation|last2=Volberg|first2=Randall|publisher=Fusion One Corporation|year=2017}}</ref> |
|||
== Fuels == |
|||
The fuels considered for fusion power have all been light elements like the isotopes of hydrogen—[[Isotopes of hydrogen#Hydrogen-1 (Protium)|protium]], [[deuterium]], and [[tritium]].<ref name="osti.gov"/> The deuterium and [[helium-3]] reaction requires helium-3, an isotope of helium so scarce on Earth that it would have to be [[Asteroid mining|mined extraterrestrially]] or produced by other nuclear reactions. Ultimately, researchers hope to adopt the protium–boron-11 reaction, because it does not directly produce neutrons, although side reactions can.<ref name="AtzeniMeyer-ter-Vehn2004">{{cite book |first1=Stefano |last1=Atzeni |first2=Jürgen |last2=Meyer-ter-Vehn |date=3 June 2004 |title=The Physics of Inertial Fusion: BeamPlasma Interaction, Hydrodynamics, Hot Dense Matter |url={{google books |plainurl=y |id=BJcy_p5pUBsC}} |publisher=OUP Oxford |pages=12–13 |isbn=978-0191524059}}</ref> |
|||
=== Deuterium, tritium === |
|||
{{main article|Deuterium–tritium fusion}} |
|||
[[File:Deuterium-tritium fusion.svg|thumb|upright=0.8|Diagram of the [[D+T|D-T]] reaction]] |
|||
The easiest nuclear reaction, at the lowest energy, is D+T: |
|||
:{{nuclide|Deuterium|link=yes}} + {{nuclide|Tritium|link=yes}} → {{nuclide|Helium|link=yes}} (3.5 MeV) + {{SubatomicParticle|10neutron|link=yes}} (14.1 MeV) |
|||
This reaction is common in research, industrial and military applications, usually as a neutron source. [[Deuterium]] is a naturally occurring [[isotope]] of hydrogen and is commonly available. The large mass ratio of the hydrogen isotopes makes their separation easy compared to the [[uranium enrichment]] process. [[Tritium]] is a natural isotope of hydrogen, but because it has a short [[half-life]] of 12.32 years, it is hard to find, store, produce, and is expensive. Consequently, the deuterium-tritium fuel cycle requires the breeding of tritium from [[lithium]] using one of the following reactions: |
|||
:{{SubatomicParticle|10neutron}} + {{nuclide|Lithium|6}} → {{nuclide|Tritium}} + {{nuclide|Helium}} |
|||
:{{SubatomicParticle|10neutron}} + {{nuclide|Lithium|7}} → {{nuclide|Tritium}} + {{nuclide|Helium}} + {{SubatomicParticle|10neutron}} |
|||
The reactant neutron is supplied by the D-T fusion reaction shown above, and the one that has the greatest energy yield. The reaction with <sup>6</sup>Li is [[exothermic reaction|exothermic]], providing a small energy gain for the reactor. The reaction with <sup>7</sup>Li is [[endothermic reaction|endothermic]], but does not consume the neutron. Neutron multiplication reactions are required to replace the neutrons lost to absorption by other elements. Leading candidate neutron multiplication materials are [[beryllium]] and [[lead]], but the <sup>7</sup>Li reaction helps to keep the neutron population high. Natural lithium is mainly <sup>7</sup>Li, which has a low tritium production [[Neutron cross section|cross section]] compared to <sup>6</sup>Li so most reactor designs use [[breeding blanket]]s with enriched <sup>6</sup>Li. |
|||
Drawbacks commonly attributed to D-T fusion power include: |
|||
* The supply of neutrons results in [[neutron activation]] of the reactor materials.<ref>{{Cite book|last1=Velarde|first1=Guillermo |title=Nuclear fusion by inertial confinement: a comprehensive treatise|last2=Martínez-Val|first2=José María|last3=Ronen|first3=Yigal|date=1993|publisher=CRC Press|isbn=978-0849369261|location=Boca Raton; Ann Arbor; London|language=en|oclc=468393053}}</ref><sup>:242</sup> |
|||
* 80% of the resultant energy is carried off by neutrons, which limits the use of direct energy conversion.<ref>{{cite journal |last1=Iiyoshi |first1=A |last2=Momota |first2=H. |last3=Motojima |first3=O. |display-authors=etal |date=October 1993 |title=Innovative Energy Production in Fusion Reactors |url=http://www.nifs.ac.jp/report/nifs250.html |url-status=dead |journal=National Institute for Fusion Science NIFS |pages=2–3 |bibcode=1993iepf.rept.....I |archive-url=https://web.archive.org/web/20150904055903/http://www.nifs.ac.jp/report/nifs250.html |archive-date=September 4, 2015 |access-date=14 February 2012}}</ref> |
|||
* It requires the [[radioisotope]] tritium. Tritium may leak from reactors. Some estimates suggest that this would represent a substantial environmental radioactivity release.<ref>{{Cite web|title=Nuclear Fusion : WNA – World Nuclear Association|url=https://www.world-nuclear.org/information-library/current-and-future-generation/nuclear-fusion-power.aspx|access-date=2020-10-11|website=www.world-nuclear.org}}</ref> |
|||
The [[neutron flux]] expected in a commercial D-T fusion reactor is about 100 times that of fission power reactors, posing problems for [[plasma facing material|material design]]. After a series of D-T tests at [[Joint European Torus|JET]], the vacuum vessel was sufficiently radioactive that it required remote handling for the year following the tests.<ref>{{cite journal|last=Rolfe|first=A. C.|title=Remote Handling JET Experience|journal=Nuclear Energy|date=1999|volume=38|issue=5|page=6|url=http://www.iop.org/Jet/fulltext/JETP99028.pdf|access-date=10 April 2012|issn=0140-4067}}</ref> |
|||
In a production setting, the neutrons would react with lithium in the breeding blanket composed of lithium ceramic pebbles or liquid lithium, yielding tritium. The energy of the neutrons ends up in the lithium, which would then be transferred to drive electrical production. The lithium blanket protects the outer portions of the reactor from the neutron flux. Newer designs, the advanced tokamak in particular, use lithium inside the reactor core as a design element. The plasma interacts directly with the lithium, preventing a problem known as "recycling". The advantage of this design was demonstrated in the [[Lithium Tokamak Experiment]]. |
|||
=== Deuterium === |
|||
[[File:Deuterium Deuterium Fusion Cross Section.png|thumbnail|upright=1.7|Deuterium fusion cross section (in square meters) at different ion collision energies]] |
|||
Fusing two deuterium nuclei is the second easiest fusion reaction. The reaction has two branches that occur with nearly equal probability: |
|||
===D-D fuel cycle=== |
|||
Though more difficult to facilitate than the deuterium-tritium reaction, fusion can also be achieved through the reaction of deuterium with itself. This reaction has two branches that occur with nearly equal probability: |
|||
:{| |
:{| |
||
| |
|D + D || → T|| + <sup>1</sup>H |
||
|- |
|- |
||
| |
|D + D || → <sup>3</sup>He || + n |
||
|} |
|} |
||
The optimum energy for this reaction is 15 keV, only slightly higher than the optimum for the D-T reaction. The first branch does not produce neutrons, but it does produce tritium, so that a D-D reactor will not be completely tritium-free, even though it does not require an input of tritium or lithium. Most of the tritium produced will be burned before leaving the reactor, which reduces the tritium handling required, but also means that more neutrons are produced and that some of these are very energetic. The neutron from the second branch has an energy of only {{convert|2.45|MeV|abbr=on}}, whereas the neutron from the D-T reaction has an energy of {{convert|14.1|MeV|abbr=on}}, resulting in a wider range of isotope production and material damage. Assuming complete tritium burn-up, the reduction in the fraction of fusion energy carried by neutrons is only about 18%, so that the primary advantage of the D-D fuel cycle is that tritium breeding is not required. Other advantages are independence from limitations of lithium resources and a somewhat softer neutron spectrum. The price to pay compared to D-T is that the energy confinement (at a given pressure) must be 30 times better and the power produced (at a given pressure and volume) is 68 times less. |
|||
This reaction is also common in research. The optimum energy to initiate this reaction is 15 keV, only slightly higher than that for the D-T reaction. The first branch produces tritium, so that a D-D reactor is not tritium-free, even though it does not require an input of tritium or lithium. Unless the tritons are quickly removed, most of the tritium produced is burned in the reactor, which reduces the handling of tritium, with the disadvantage of producing more, and higher-energy, neutrons. The neutron from the second branch of the D-D reaction has an energy of only {{convert|2.45|MeV|abbr=on}}, while the neutron from the D-T reaction has an energy of {{convert|14.1|MeV|abbr=on}}, resulting in greater isotope production and material damage. When the tritons are removed quickly while allowing the <sup>3</sup>He to react, the fuel cycle is called "tritium suppressed fusion".<ref>{{Cite journal |last1=Sawan |first1=M. E. |last2=Zinkle |first2=S. J. |last3=Sheffield |first3=J. |date=2002 |title=Impact of tritium removal and He-3 recycling on structure damage parameters in a D–D fusion system |url=http://dx.doi.org/10.1016/s0920-3796(02)00104-7 |journal=Fusion Engineering and Design |volume=61–62 |pages=561–567 |doi=10.1016/s0920-3796(02)00104-7 |bibcode=2002FusED..61..561S |issn=0920-3796}}</ref> The removed tritium decays to <sup>3</sup>He with a 12.5 year half life. By recycling the <sup>3</sup>He decay into the reactor, the fusion reactor does not require materials resistant to fast neutrons. |
|||
===D-<sup>3</sup>He fuel cycle=== |
|||
A second-generation approach to controlled fusion power involves combining [[helium-3]] (<sup>3</sup>He) and deuterium (<sup>2</sup>H). This reaction produces a helium-4 nucleus (<sup>4</sup>He) and a high-energy proton. As with the p-<sup>11</sup>B [[aneutronic fusion]] fuel cycle, most of the reaction energy is released as charged particles, reducing activation of the reactor housing and potentially allowing more efficient energy harvesting (via any of several speculative technologies). In practice, D-D side reactions produce a significant number of neutrons, resulting in p-<sup>11</sup>B being the preferred cycle for aneutronic fusion. |
|||
Assuming complete tritium burn-up, the reduction in the fraction of fusion energy carried by neutrons would be only about 18%, so that the primary advantage of the D-D fuel cycle is that tritium breeding is not required. Other advantages are independence from lithium resources and a somewhat softer neutron spectrum. The disadvantage of D-D compared to D-T is that the energy confinement time (at a given pressure) must be 30 times longer and the power produced (at a given pressure and volume) is 68 times less.{{Citation needed|date=November 2014}} |
|||
===p-<sup>11</sup>B fuel cycle=== |
|||
If [[aneutronic fusion]] is the goal, then the most promising candidate may be the Hydrogen-1 (proton)/[[boron]] reaction: |
|||
:<sup>1</sup>H + <sup>11</sup>B → 3 <sup>4</sup>He |
|||
Under reasonable assumptions, side reactions will result in about 0.1% of the fusion power being carried by neutrons.<ref>Heindler and Kernbichler, Proc. 5th Intl. Conf. on Emerging Nuclear Energy Systems, 1989, pp. 177-82. See also [[Aneutronic fusion#Residual radiation from a p–11B reactor|Residual radiation from a p–11B reactor]]</ref> At 123 keV, the optimum temperature for this reaction is nearly ten times higher than that for the pure hydrogen reactions, the energy confinement must be 500 times better than that required for the D-T reaction, and the [[power density]] will be 2500 times lower than for D-T. Since the confinement properties of conventional approaches to fusion such as the tokamak and laser pellet fusion are marginal, most proposals for aneutronic fusion are based on radically different confinement concepts, such as the [[Polywell]] and the [[Dense plasma focus]]. |
|||
Assuming complete removal of tritium and <sup>3</sup>He recycling, only 6% of the fusion energy is carried by neutrons. The tritium-suppressed D-D fusion requires an energy confinement that is 10 times longer compared to D-T and double the plasma temperature.<ref>J. Kesner, D. Garnier, A. Hansen, M. Mauel, and L. Bromberg, ''Nucl Fusion'' 2004; 44, 193</ref> |
|||
==History of research== |
|||
===Brief overview=== |
|||
The idea of using human-initiated fusion reactions was first made practical for military purposes in [[nuclear weapon]]s. In a hydrogen bomb, the energy released by a fission weapon is used to compress and heat fusion fuel, beginning a fusion reaction that releases a large amount of neutrons that increases the rate of fission. The first [[Fusion-boosted fission weapon|fission-fusion-fission-based]] weapons released some 500 times more energy than early fission weapons. |
|||
=== Deuterium, helium-3 === |
|||
Attempts at controlling fusion had already started by this point. Registration of the first patent related to a fusion reactor<ref>[http://v3.espacenet.com/textdoc?DB=EPODOC&IDX=GB817681&F=0 British Patent 817681, available here]</ref> by the [[United Kingdom Atomic Energy Authority]], the inventors being [[George Paget Thomson|Sir George Paget Thomson]] and [[Moses Blackman]], dates back to 1946. This was the first detailed examination of the pinch concept, and small efforts to experiment with the pinch concept started at several sites in the UK. |
|||
A second-generation approach to controlled fusion power involves combining [[helium-3]] (<sup>3</sup>He) and [[deuterium]] (<sup>2</sup>H): |
|||
:{| |
|||
Around the same time, an expatriate German proposed the [[Huemul Project]] in Argentina, announcing positive results in 1951. Although these results turned out to be false, it sparked off intense interest around the world. The UK pinch programs were greatly expanded, culminating in the [[ZETA]] and [[Sceptre (fusion reactor)|Sceptre]] devices. In the US, pinch experiments like those in the UK started at the [[Los Alamos National Laboratory]]. Similar devices were built in the USSR after data on the UK program was passed to them by [[Klaus Fuchs]]. At [[Princeton University]] a new approach developed as the [[stellarator]], and the research establishment formed there continues to this day as the [[Princeton Plasma Physics Laboratory]]. Not to be outdone, [[Lawrence Livermore National Laboratory]] entered the field with their own variation, the [[magnetic mirror]]. These three groups have remained the primary developers of fusion research in the US to this day. |
|||
|D + <sup>3</sup>He || → <sup>4</sup>He || + <sup>1</sup>H |
|||
|} |
|||
This reaction produces <sup>4</sup>He and a high-energy proton. As with the p-<sup>11</sup>B [[aneutronic fusion]] fuel cycle, most of the reaction energy is released as charged particles, reducing [[neutron activation|activation]] of the reactor housing and potentially allowing more efficient energy harvesting (via any of several pathways).<ref name="Advanced Fuels">{{Cite journal|last=Nevins|first=W. M.|date=1998-03-01|title=A Review of Confinement Requirements for Advanced Fuels|url=https://doi.org/10.1023/A:1022513215080|journal=Journal of Fusion Energy|language=en|volume=17|issue=1|pages=25–32|doi=10.1023/A:1022513215080|bibcode=1998JFuE...17...25N|s2cid=118229833|issn=1572-9591}}</ref> In practice, D-D side reactions produce a significant number of neutrons, leaving p-<sup>11</sup>B as the preferred cycle for aneutronic fusion.<ref name="Advanced Fuels" /> |
|||
In the time since these early experiments, two new approaches developed that have since come to dominate fusion research. The first was the [[tokamak]] approach developed in the Soviet Union, which combined features of the stellarator and pinch to produce a device that dramatically outperformed either. The majority of magnetic fusion research to this day has followed the tokamak approach. In the late 1960s the concept of "mechanical" fusion through the use of [[laser]]s was developed in the US, and Lawrence Livermore switched their attention from mirrors to lasers over time. |
|||
=== Proton, boron-11 === |
|||
Civilian applications are still being developed. Although it took less than ten years for fission to go from military applications to civilian fission energy production,<ref>The first A-bomb shot dates back to July 16, 1945 in [[Alamogordo]] ([[New Mexico]] desert), while the first civilian fission plant was connected to the electric power network on June 27, 1954 in [[Obninsk]] ([[Russia]]).</ref> it has been very different in the fusion energy field; more than fifty years have already passed since the first fusion reaction took place<ref>The first H-bomb, [[Ivy Mike]], was detonated on [[Enewetak|Eniwetok]], an atoll of the [[Pacific Ocean]], on November 1, 1952 (local time).</ref> and sixty years since the first attempts to produce controlled fusion power, without any commercial fusion energy production plant coming into operation. |
|||
Both material science problems and non-proliferation concerns are greatly diminished by [[aneutronic fusion]]. Theoretically, the most reactive aneutronic fuel is <sup>3</sup>He. However, obtaining reasonable quantities of <sup>3</sup>He implies large scale extraterrestrial mining on the Moon or in the atmosphere of Uranus or Saturn. Therefore, the most promising candidate fuel for such fusion is fusing the readily available protium (i.e. a [[proton]]) and [[boron]]. Their fusion releases no neutrons, but produces energetic charged alpha (helium) particles whose energy can directly be converted to electrical power: |
|||
: p + <sup>11</sup>B → 3 <sup>4</sup>He |
|||
=== Magnetic containment === |
|||
====Pinch devices==== |
|||
[[Image:Wires array.jpg|thumb|right|300px|A "wires array" used in [[Z-pinch]] confinement, during the building process]] |
|||
A major area of study in early fusion power research is the "[[Pinch (plasma physics)|pinch]]" concept. Pinch is based on the fact the plasmas are electrically conducting. By running a current through the plasma, a magnetic field will be generated around the plasma. This field will, according to [[Lenz's law]], create an inward directed force that causes the plasma to collapse inward, raising its density. Denser plasmas generate denser magnetic fields, increasing the inward force, leading to a [[chain reaction]]. If the conditions are correct, this can lead to the densities and temperatures needed for fusion. The trick is getting the current into the plasma; this is solved by inducing the current from an external magnet, which also produces the external field the internal field acts against. |
|||
Side reactions are likely to yield neutrons that carry only about 0.1% of the power,<ref>{{Cite book |title=Emerging nuclear energy systems 1989: proceedings of the Fifth International Conference on Emerging Nuclear Energy Systems, Karlsruhe, F.R. Germany, July 3–6, 1989|date=1989|publisher=World Scientific |editor=von Möllendorff, Ulrich |editor2=Goel, Balbir |isbn=981-0200102|location=Singapore|oclc=20693180}}</ref><sup>:177–182</sup> which means that [[neutron scattering]] is not used for energy transfer and material activation is reduced several thousand-fold. The optimum temperature for this reaction of 123 keV<ref>{{Cite journal|last1=Feldbacher|first1=Rainer|last2=Heindler|first2=Manfred|date=1988|title=Basic cross section data for aneutronic reactor |journal=Nuclear Instruments and Methods in Physics Research Section A: Accelerators, Spectrometers, Detectors and Associated Equipment|volume=271|issue=1|pages=55–64|doi=10.1016/0168-9002(88)91125-4|bibcode=1988NIMPA.271...55F|issn=0168-9002}}</ref> is nearly ten times higher than that for pure hydrogen reactions, and energy confinement must be 500 times better than that required for the D-T reaction. In addition, the [[power density]] is 2500 times lower than for D-T, although per unit mass of fuel, this is still considerably higher compared to fission reactors. |
|||
Pinch was first developed in the UK in the immediate post-war era. Starting in 1947 small experiments were carried out and plans were laid to build a much larger machine. When the Huemul results hit the news, [[James L. Tuck]], a UK physicist working at Los Alamos, introduced the pinch concept in the US and produced a series of machines known as the [[Perhapsatron]]. In the Soviet Union, a series of similar machines were being built, unknown in the west. All of these devices quickly demonstrated a series of instabilities in the fusion when the pinch was applied, which broke up the plasma column long before it reached the densities and temperatures needed for fusion. In 1953 Tuck and others suggested a number of solutions to these problems. |
|||
Because the confinement properties of the tokamak and laser pellet fusion are marginal, most proposals for aneutronic fusion are based on radically different confinement concepts, such as the [[Polywell]] and the [[Dense Plasma Focus]]. In 2013, a research team led by [[Christine Labaune]] at [[École Polytechnique]], reported a new fusion rate record for proton-boron fusion, with an estimated 80 million fusion reactions during a 1.5 nanosecond laser fire, 100 times greater than reported in previous experiments.<ref>{{cite web|url=http://www.livescience.com/40246-new-boron-method-nuclear-fusion.html|title=Nuclear Fusion: Laser-Beam Experiment Yields Exciting Results|website=LiveScience.com|date=October 8, 2013}}</ref><ref>{{cite web|url=http://www.fusenet.eu/node/575|title=Record proton-boron fusion rate achieved – FuseNet|website=www.fusenet.eu|access-date=2014-11-26|archive-url=https://web.archive.org/web/20141202062802/http://www.fusenet.eu/node/575|archive-date=2014-12-02|url-status=dead}}</ref> |
|||
The largest "classic" pinch device was the [[ZETA]], including all of these upgrades, starting operations in the UK in 1957. In early 1958 [[John Cockcroft]] announced that fusion had been achieved in the ZETA, an announcement that made headlines around the world. When physicists in the US expressed concerns about the claims they were initially dismissed. However, US experiments demonstrated the same neutrons, although measurements suggested these could not be from fusion reactions. The neutrons seen in the UK were later demonstrated to be from different versions of the same instability processes that plagued earlier machines. Cockcroft was forced to retract the fusion claims, which tainted the entire field for years. ZETA ended its experiments in 1968, and most other pinch experiments ended shortly after. |
|||
== Material selection == |
|||
In 1974 a study of the ZETA results demonstrated an interesting side-effect; after the experimental runs ended, the plasma would enter a short period of stability. This led to the [[reversed field pinch]] concept which has seen some level of development ever since. Recent work on the basic concept started as a result of the appearance of the "wires array" concept in the 1980s, which allowed a more efficient use of this technique. The [[Sandia National Laboratory]] runs a continuing wire-array research program with the [[Z machine|Zpinch]] machine. In addition, the [[University of Washington]]'s [http://www.aa.washington.edu/AERP/ZaP/ ZaP Lab] has shown quiescent periods of stability hundreds of times longer than expected for plasma in a Z-pinch configuration, giving promise to the confinement technique. |
|||
{{Further|International Fusion Materials Irradiation Facility}} |
|||
Structural material stability is a critical issue.<ref name="Materials">{{Cite book|last=Roberts, J. T. Adrian |title=Structural Materials in Nuclear Power Systems|date=1981|publisher=Springer US|isbn=978-1468471960|location=Boston, MA|oclc=853261260}}</ref><ref>{{Cite web|date=2021-09-09|title=Roadmap highlights materials route to fusion|url=https://www.theengineer.co.uk/fusion-materials/|access-date=2021-09-17|website=The Engineer|language=en-US}}</ref> Materials that can survive the high temperatures and neutron bombardment experienced in a fusion reactor are considered key to success.<ref>{{Cite journal |last=Klueh |first=R. L. |title=Metals in the nuclear-fusion environment |journal=Materials Engineering |volume=99 |pages=39–42}}</ref><ref name="Materials" /> The principal issues are the conditions generated by the plasma, neutron degradation of wall surfaces, and the related issue of plasma-wall surface conditions.<ref>{{Cite thesis|title=Interaction of atomic hydrogen with materials used for plasma-facing wall in fusion devices |type=Doctorate |publisher=[A. Založnik]|date=2016|place=Ljubljana|language=en|first=Anže|last=Založnik|oclc = 958140759}}</ref><ref>{{Cite journal |last=McCracken |first=G. M. |date=1997 |title=Plasma surface interactions in controlled fusion devices |url=http://dx.doi.org/10.1088/0029-5515/37/3/413 |journal=Nuclear Fusion |volume=37 |issue=3 |pages=427–429 |doi=10.1088/0029-5515/37/3/413 |issn=0029-5515 |s2cid=250776874}}</ref> Reducing hydrogen permeability is seen as crucial to hydrogen recycling<ref>{{Citation|last=Mioduszewski|first=Peter|title=Hydrogen Recycling and Wall Equilibration in Fusion Devices |date=2000|url=http://dx.doi.org/10.1007/978-94-011-4331-8_23|work=Hydrogen Recycling at Plasma Facing Materials|pages=195–201|place=Dordrecht|publisher=Springer Netherlands|doi=10.1007/978-94-011-4331-8_23|isbn=978-0792366300|access-date=2020-10-13}}</ref> and control of the tritium inventory.<ref name="permeation">{{Cite journal|last=Nemanič|first=Vincenc|date=2019|title=Hydrogen permeation barriers: Basic requirements, materials selection, deposition methods, and quality evaluation|journal=Nuclear Materials and Energy|volume=19|pages=451–457|doi=10.1016/j.nme.2019.04.001|issn=2352-1791|doi-access=free|bibcode=2019NMEne..19..451N }}</ref> Materials with the lowest bulk hydrogen solubility and diffusivity provide the optimal candidates for stable barriers. A few pure metals, including tungsten and beryllium,<ref>{{cite web|url=https://www.americanelements.com/fusion-energy|title=American Elements Creates Detection Window for EPFL Fusion Reactor|access-date=16 February 2023|publisher=American Elements}}</ref> and compounds such as carbides, dense oxides, and nitrides have been investigated. Research has highlighted that coating techniques for preparing well-adhered and perfect barriers are of equivalent importance. The most attractive techniques are those in which an ad-layer is formed by oxidation alone. Alternative methods utilize specific gas environments with strong magnetic and electric fields. Assessment of barrier performance represents an additional challenge. Classical coated membranes gas permeation continues to be the most reliable method to determine hydrogen permeation barrier (HPB) efficiency.<ref name="permeation" /> In 2021, in response to increasing numbers of designs for fusion power reactors for 2040, the [[United Kingdom Atomic Energy Authority]] published the [https://www.royce.ac.uk/content/uploads/2021/09/UK_Fusion_Materials_Roadmap_Interactive.pdf UK Fusion Materials Roadmap 2021–2040], focusing on five priority areas, with a focus on tokamak family reactors: |
|||
In 1995, the staged Z-pinch concept was introduced by a team of scientist from University of California Irvine (UCI). This scheme can control one of the most dangerous instability that normally disintegrate conventional Z-pinch before the final implosion. The concept is based on a complex load of radiative liner plasma embedded with a target plasma. During implosion the outer surface of the liner plasma becomes unstable but the target plasma remains remarkably stable, up until the final implosion, generating a very high energy density stable target plasma. The heating mechanisms are shock heating, adiabatic compression and trapping of charge particles produced in fusion reaction due to a very strong magnetic field, which develops between the liner and the target. Details of this concept are shown in various publications available on the web page of MIFTI [http://www.mifti.com]. |
|||
* Novel materials to minimize the amount of activation in the structure of the fusion power plant; |
|||
====Early magnetic approaches==== |
|||
* Compounds that can be used within the power plant to optimise breeding of tritium fuel to sustain the fusion process; |
|||
The U.S. fusion program began in 1951 when [[Lyman Spitzer]] began work on a [[stellarator]] under the code name Project Matterhorn. His work led to the creation of the [[Princeton Plasma Physics Laboratory]], where magnetically confined plasmas are still studied. Spitzer planned an aggressive development project of four machines, A, B, C, and D. A and B were small research devices, C would be the prototype of a power-producing machine, and D would be the prototype of a commercial device. A worked without issue, but even by the time B was being used it was clear the stellarator was also suffering from instabilities and plasma leakage. Progress on C slowed as attempts were made to correct for these problems. |
|||
* Magnets and insulators that are resistant to irradiation from fusion reactions—especially under cryogenic conditions; |
|||
* Structural materials able to retain their strength under neutron bombardment at high operating temperatures (over 550 degrees C); |
|||
* Engineering assurance for fusion materials—providing irradiated sample data and modelled predictions such that plant designers, operators and regulators have confidence that materials are suitable for use in future commercial power stations. |
|||
=== Superconducting materials === |
|||
At Lawrence Livermore, the [[magnetic mirror]] was the preferred approach. The mirror consisted of two large magnets arranged so they had strong fields within them, and a weaker, but connected, field between them. Plasma introduced in the area between the two magnets would "bounce back" from the stronger fields in the middle. Although the design would leak plasma through the mirrors, the rate of leakage would be low enough that a useful fusion rate could be maintained. The simplicity of the design was supposed to make up for its lower performance. In practice the mirror also suffered from mysterious leakage problems, and never reached the expected performance. |
|||
[[File:SuperOX Wire Production from 2013 to 2021.png|thumbnail|upright=1.5|SuperOx was able to produce over 186 miles of YBCO wire in nine months for use in fusion reactor magnets, dramatically surpassing the company's previous production targets.]] |
|||
In a plasma that is embedded in a magnetic field (known as a magnetized plasma) the fusion rate scales as the magnetic field strength to the 4th power. For this reason, many fusion companies that rely on magnetic fields to control their plasma are trying to develop high temperature superconducting devices. In 2021, SuperOx, a Russian and Japanese company, developed a new manufacturing process for making superconducting [[YBCO]] wire for fusion reactors. This new wire was shown to conduct between 700 and 2000 Amps per square millimeter. The company was able to produce 186 miles of wire in nine months.<ref>Molodyk, A., et al. "Development and large volume production of extremely high current density YBa2Cu3O7 superconducting wires for fusion." ''Scientific Reports'' 11.1 (2021): 1–11.</ref> |
|||
====Gun Club, MHD, instability; progress slows==== |
|||
By the mid-1950s it was clear that the simple theoretical tools being used to calculate the performance of all fusion machines were simply not predicting their actual behaviour. Machines invariably leaked their plasma from their confinement area at rates far higher than predicted. |
|||
=== Containment considerations === |
|||
In 1954, [[Edward Teller]] held a gathering of fusion researchers at the Princeton Gun Club, near the Project Matterhorn (now known as Project Sherwood) grounds. Teller started by pointing out the problems that everyone was having, and suggested that any system where the plasma was confined within concave fields was doomed to fail. Attendees remember him saying something to the effect that the fields were like rubber bands, and they would attempt to snap back to a straight configuration whenever the power was increased, ejecting the plasma. He went on to say that it appeared the only way to confine the plasma in a stable configuration would be to use convex fields, a "cusp" configuration.<ref>Nathaniel Fisch, [http://books.google.com/books?id=9i9bgMLVjWsC&pg=PA118 "Edward Teller Centennial Symposium"], pg 118</ref> |
|||
Even on smaller production scales, the containment apparatus is blasted with matter and energy. Designs for plasma containment must consider: |
|||
* A heating and cooling cycle, up to a 10 MW/m<sup>2</sup> thermal load. |
|||
When the meeting concluded, most of the researchers quickly turned out papers saying why Teller's concerns did not apply to their particular device. The pinch machines did not use magnetic fields in this way at all, while the mirror and stellarator seemed to have various ways out. However, this was soon followed by a paper by [[Martin David Kruskal]] and [[Martin Schwarzschild]] discussing pinch machines, which demonstrated instabilities in those devices were inherent to the design. A series of similar studies followed, abandoning the simplistic theories previously used and introducing a full consideration of [[magnetohydrodynamics]] with a partially-resistive plasma. These concepts developed quickly, and by the early 1960s it was clear that small devices simply would not work. A series of much larger and more complex devices followed as researchers attempted to add field upon field in order to provide the required field strength without reaching the unstable regimes. As cost and complexity climbed, the initial optimism of the fusion field faded. |
|||
* [[Neutron radiation]], which over time leads to [[neutron activation]] and [[embrittlement]]. |
|||
* High energy ions leaving at tens to hundreds of [[electronvolt]]s. |
|||
* [[Alpha particle]]s leaving at millions of [[electronvolt]]s. |
|||
* Electrons leaving at high energy. |
|||
* Light radiation (IR, visible, UV, X-ray). |
|||
Depending on the approach, these effects may be higher or lower than fission reactors.<ref name="Shin Kajita 2014">"Thermal response of nanostructured tungsten". Shin Kajita, et al., January 2014, Nuclear Fusion 54 (2014) 033005 (10 pp.)</ref> One estimate put the [[neutron radiation|radiation]] at 100 times that of a typical [[pressurized water reactor]].{{Citation needed|date=March 2014}} Depending on the approach, other considerations such as [[electrical conductivity]], [[Vacuum permeability|magnetic permeability]], and mechanical strength matter. Materials must also not end up as long-lived [[radioactive waste]].<ref name="Materials" /> |
|||
====The tokamak is announced==== |
|||
A new approach was outlined in the theoretical works fulfilled in 1950–1951 by [[Igor Tamm|I.E. Tamm]] and [[Andrei Sakharov|A.D. Sakharov]] in the [[Soviet Union]], which first discussed a [[tokamak]]-like approach. Experimental research on these designs began in 1956 at the [[Kurchatov Institute]] in [[Moscow]] by a group of Soviet scientists led by [[Lev Artsimovich]]. The tokamak essentially combined a low-power pinch device with a low-power simple stellarator. The key was to combine the fields in such a way that the particles wound around the reactor a particular number of times, today known as the "[[Safety factor (plasma physics)|safety factor]]". The combination of these fields dramatically improved confinement times and densities, resulting in huge improvements over existing devices. |
|||
=== Plasma-wall surface conditions === |
|||
The group constructed the first tokamaks, the most successful being the [[T-3 (tokamak)|T-3]] and its larger version [[T-4 (tokamak)|T-4]]. T-4 was tested in 1968 in [[Novosibirsk]], producing the first quasistationary thermonuclear fusion reaction ever.<ref>[[Great Soviet Encyclopedia]], 3rd edition, entry on "Токамак", available online [http://slovari.yandex.ru/art.xml?art=bse/00079/49400.htm here]</ref> The tokamak was dramatically more efficient than the other approaches of that era, on the order of 10 to 100 times. When they were first announced the international community was highly skeptical. However, a British team was invited to see T-3, and having measured it in depth they released their results that confirmed the Soviet claims. A burst of activity followed as many planned devices were abandoned and new tokamaks were introduced in their place - the C model stellarator, then under construction after many redesigns, was quickly converted to the [[Symmetrical Tokamak]] and the stellarator was abandoned. |
|||
For long term use, each atom in the wall is expected to be hit by a neutron and displaced about 100 times before the material is replaced. High-energy neutrons produce hydrogen and helium via nuclear reactions that tend to form bubbles at grain boundaries and result in swelling, blistering or embrittlement.<ref name="Shin Kajita 2014"/> |
|||
=== Selection of materials === |
|||
Through the 1970s and 80s great strides in understanding the tokamak system were made. A number of improvements to the design are now part of the "advanced tokamak" concept, which includes non-circular plasmas, internal diverters and limiters, often superconducting magnets, and operate in the so-called "[[H-mode]]" island of increased stability. Two other designs have also become fairly well studied; the compact tokamak is wired with the magnets on the inside of the vacuum chamber, while the [[spherical tokamak]] reduces its cross section as much as possible. |
|||
Low-[[Atomic number|Z]] materials, such as [[graphite]] or [[beryllium]] are generally preferred to high-Z materials, usually [[tungsten]] with [[molybdenum]] as a second choice.<ref name="permeation" /> Liquid metals (lithium, [[gallium]], [[tin]]) have been proposed, e.g., by injection of 1–5 mm thick streams flowing at 10 m/s on solid substrates.{{Citation needed|date=March 2014}} |
|||
Graphite features a gross erosion rate due to physical and chemical [[sputtering]] amounting to many meters per year, requiring redeposition of the sputtered material. The redeposition site generally does not exactly match the sputter site, allowing net erosion that may be prohibitive. An even larger problem is that tritium is redeposited with the redeposited graphite. The tritium inventory in the wall and dust could build up to many kilograms, representing a waste of resources and a radiological hazard in case of an accident. Graphite found favor as material for short-lived experiments, but appears unlikely to become the primary [[plasma-facing material]] (PFM) in a commercial reactor.<ref name="Materials" /><ref>{{Cite journal|title= Plasma–surface interaction in the stellarator W7-X: Conclusions drawn from operation with graphite plasma-facing components|journal=Nuclear Fusion|date=December 2, 2021 |volume=62 |issue=1 |page=016006 |doi=10.1088/1741-4326/ac3508 |s2cid=240484560 |last1=Brezɩnsek |first1=S. |last2=Dhard |first2=C. P. |last3=Jakubowski |first3=M. |last4=König |first4=R. |last5=Masuzaki |first5=S. |last6=Mayer |first6=M. |last7=Naujoks |first7=D. |last8=Romazanov |first8=J. |last9=Schmid |first9=K. |last10=Schmitz |first10=O. |last11=Zhao |first11=D. |last12=Balden |first12=M. |last13=Brakel |first13=R. |last14=Butterschoen |first14=B. |last15=Dittmar |first15=T. |last16=Drews |first16=P. |last17=Effenberg |first17=F. |last18=Elgeti |first18=S. |last19=Ford |first19=O. |last20=Fortuna-Zalesna |first20=E. |last21=Fuchert |first21=G. |last22=Gao |first22=Y. |last23=Goriaev |first23=A. |last24=Hakola |first24=A. |last25=Kremeyer |first25=T. |last26=Krychowiak |first26=M. |last27=Liang |first27=Y. |last28=Linsmeier |first28=Ch |last29=Lunsford |first29=R. |last30=Motojima |first30=G. |display-authors=1 |doi-access=free }}</ref> |
|||
The tokamak dominates modern research, where very large devices like [[ITER]] are expected to pass several milestones toward commercial power production, including a [[Fusion energy gain factor|burning plasma]] with long burn times, high power output, and online fueling. There are no guarantees that the project will be successful; previous generations of tokamak machines have uncovered new problems many times. But the entire field of high temperature plasmas is much better understood now than formerly, and there is considerable optimism that ITER will meet its goals. If successful, ITER would be followed by a "[[DEMO|commercial demonstrator]]" system, similar in purpose to the very earliest power-producing fission reactors built in the era before wide-scale commercial deployment of larger machines started in the 1960s and 1970s. |
|||
Tungsten's sputtering rate is orders of magnitude smaller than carbon's, and tritium is much less incorporated into redeposited tungsten. However, tungsten plasma impurities are much more damaging than carbon impurities, and self-sputtering can be high, requiring the plasma in contact with the tungsten not be too hot (a few tens of eV rather than hundreds of eV). Tungsten also has issues around eddy currents and melting in off-normal events, as well as some radiological issues.<ref name="Materials" /> |
|||
Even with these goals met, there are a number of major engineering problems remaining, notably finding suitable "low activity" materials for reactor construction, demonstrating secondary systems including practical [[tritium]] extraction, and building reactor designs that allow their reactor core to be removed when its materials becomes embrittled due to the neutron flux. Practical commercial generators based on the tokamak concept are far in the future. The public at large has been disappointed, as the initial outlook for practical fusion power plants was much rosier; a pamphlet from the 1970s printed by General Atomic stated that "Several commercial fusion reactors are expected to be online by the year 2000." |
|||
== Safety and the environment == |
|||
===Inertial (laser) containment=== |
|||
=== Accident potential === |
|||
The technique of implosion of a microcapsule irradiated by [[laser]] beams, the basis of [[laser inertial confinement]], was first suggested in 1962 by scientists at [[Lawrence Livermore National Laboratory]], shortly after the invention of the laser itself in 1960. Lasers of the era were very low powered, but low-level research using them nevertheless started as early as 1965. A great advance in the field was John Nuckolls' 1972 paper that ignition would require lasers of about 1 kJ, and efficient burn around 1 MJ. kJ lasers were just beyond the state of the art at the time, and his paper sparked off a tremendous development effort to produce devices of the needed power. |
|||
Accident potential and effect on the environment are critical to social acceptance of nuclear fusion, also known as a [[Corporate social responsibility|social license]].<ref>{{Cite journal |last=Hoedl |first=Seth A. |date=2022 |title=Achieving a social license for fusion energy |url=http://dx.doi.org/10.1063/5.0091054 |journal=Physics of Plasmas |volume=29 |issue=9 |pages=092506 |doi=10.1063/5.0091054 |bibcode=2022PhPl...29i2506H |s2cid=252454077 |issn=1070-664X}}</ref> Fusion reactors are not subject to [[Nuclear meltdown|catastrophic meltdown]].<ref name="McCrackenStott2012"/> It requires precise and controlled temperature, pressure and magnetic field parameters to produce net energy, and any damage or loss of required control would rapidly quench the reaction.<ref name=afraid>{{cite web |url=http://www.iter.org/newsline/107/1489 |title=Who is afraid of ITER? |first=Krista |last=Dulon |website=iter.org |date=2012 |access-date=18 August 2012 |archive-url=https://web.archive.org/web/20121130221734/http://www.iter.org/newsline/107/1489 |archive-date=November 30, 2012 |url-status=dead }}</ref> Fusion reactors operate with seconds or even microseconds worth of fuel at any moment. Without active refueling, the reactions immediately quench.<ref name="McCrackenStott2012">{{cite book|last1=McCracken|first1=Garry |last2=Stott|first2=Peter |title=Fusion: The Energy of the Universe|url={{google books|plainurl=y|id=e6jEZfO2gO4C|page=198}}|access-date=18 August 2012|date=2012|publisher=Academic Press|isbn=978-0123846563|pages=198–199}}</ref> |
|||
The same constraints prevent runaway reactions. Although the plasma is expected to have a volume of {{Convert|1000|m3|ft3|abbr=on}} or more, the plasma typically contains only a few grams of fuel.<ref name="McCrackenStott2012" /> By comparison, a fission reactor is typically loaded with enough fuel for months or years, and no additional fuel is necessary to continue the reaction. This large fuel supply is what offers the possibility of a meltdown.<ref name="n">{{cite book|last=Angelo|first=Joseph A. |title=Nuclear Technology|url={{google books|plainurl=y|id=ITfaP-xY3LsC|page=474}}|access-date=18 August 2012|date=2004|publisher=Greenwood Publishing Group|isbn=978-1573563369|page=474}}</ref> |
|||
Early machines used a variety of approaches to attack one of two problems - some focused on fast delivery of energy, while others were more interested in beam smoothness. Both were attempts to ensure the energy delivery would be smooth enough to cause an even implosion. However, these experiments demonstrated a serious problem; laser wavelengths in the [[infrared]] area lost a tremendous amount of energy before compressing the fuel. Important breakthroughs in this laser technology were made at the [[Laboratory for Laser Energetics]] at the University of Rochester, where scientists used frequency-tripling crystals to transform the infrared laser beams into ultraviolet beams. By the late 1970s great strides had been made in laser power, but with each increase new problems were found in the implosion technique that suggested even more power would be required. By the 1980s these increases were so large that using the concept for generating net energy seemed remote. Most research in this field turned to weapons research, always a second line of research, as the implosion concept is somewhat similar to [[hydrogen bomb]] operation. Work on very large versions continued as a result, with the very large [[National Ignition Facility]] in the US and [[Laser Mégajoule]] in France supporting these research programs. |
|||
In magnetic containment, strong fields develop in coils that are mechanically held in place by the reactor structure. Failure of this structure could release this tension and allow the magnet to "explode" outward. The severity of this event would be similar to other industrial accidents or an [[MRI]] machine quench/explosion, and could be effectively contained within a [[containment building]] similar to those used in fission reactors. |
|||
More recent work had demonstrated that significant savings in the required laser energy are possible using a technique known as "fast ignition". The savings are so dramatic that the concept appears to be a useful technique for energy production again, so much so that it is a serious contender for pre-commercial development. There are proposals to build an experimental facility dedicated to the fast ignition approach, known as [[HiPER]]. At the same time, advances in [[solid state laser]]s appear to improve the "driver" systems' efficiency by about ten times (to 10- 20%), savings that make even the large "traditional" machines almost practical, and might make the fast ignition concept outpace the magnetic approaches in further development. |
|||
In laser-driven inertial containment the larger size of the reaction chamber reduces the stress on materials. Although failure of the reaction chamber is possible, stopping fuel delivery prevents catastrophic failure.<ref name="economic prospects">{{Cite book |title=Safety, environmental impact, and economic prospects of nuclear fusion|date=1990|publisher=Plenum Press|editor=Brunelli, B. |editor2=Knoepfel, Heinz |isbn=978-1461306191|location=New York|oclc=555791436}}</ref> |
|||
The laser-based concept has other advantages. The reactor core is mostly exposed, as opposed to being wrapped in a huge magnet as in the tokamak. This makes the problem of removing energy from the system somewhat simpler, and should mean that a laser-based device would be much easier to perform maintenance on, such as core replacement. Additionally, the lack of strong magnetic fields allows for a wider variety of low-[[Neutron activation|activation]] materials, including [[carbon fiber]], which would reduce both the frequency of such neutron activations and the rate of irradiation to the core. In other ways the program has many of the same problems as the tokamak; practical methods of energy removal and tritium recycling need to be demonstrated. |
|||
Most reactor designs rely on [[liquid hydrogen]] as a coolant and to convert stray neutrons into [[tritium]], which is fed back into the reactor as fuel. Hydrogen is flammable, and it is possible that hydrogen stored on-site could ignite. In this case, the tritium fraction of the hydrogen would enter the atmosphere, posing a radiation risk. Calculations suggest that about {{convert|1|kg}} of tritium and other radioactive gases in a typical power station would be present. The amount is small enough that it would dilute to legally acceptable limits by the time they reached the station's [[perimeter fence]].<ref name="WorldEnergyCouncil" /> |
|||
===Other approaches=== |
|||
Over the years there have been a wide variety of fusion concepts. In general they fall into three groups - those that attempt to reach high temperature/density for brief times (pinch, inertial confinement), those that operate at a steady state (magnetic confinement) or those that try neither and instead attempt to produce low quantities of fusion but do so at an extremely low cost. The latter group has largely disappeared, as the difficulties of achieving fusion have demonstrated that any low-energy device is unlikely to produce net gain. This leaves the two major approaches, magnetic and laser inertial, as the leading systems for development funding. However, alternate approaches continue to be developed, and alternate non-power fusion devices have been successfully developed as well. |
|||
The likelihood of small industrial accidents, including the local release of radioactivity and injury to staff, are estimated to be minor compared to fission. They would include accidental releases of lithium or tritium or mishandling of radioactive reactor components.<ref name="economic prospects" /> |
|||
====Technically viable==== |
|||
[[Philo T. Farnsworth]], the inventor of the first [[History of television|all-electronic television system]] in 1927, patented his first [[Fusor]] design in 1968, a device that uses [[inertial electrostatic confinement]]. This system consists largely of two concentric spherical electrical grids inside a vacuum chamber into which a small amount of fusion fuel is introduced. Voltage across the grids causes the fuel to ionize around them, and positively charged ions are accelerated towards the center of the chamber. Those ions may collide and fuse with ions coming from the other direction, may scatter without fusing, or may pass directly through. In the latter two cases, the ions will tend to be stopped by the electric field and re-accelerated toward the center. Fusors can also use ion guns rather than electric grids. Towards the end of the 1960s, [[Robert L. Hirsch|Robert Hirsch]] designed a variant of the Farnsworth Fusor known as the [[Hirsch-Meeks fusor]]. This variant is a considerable improvement over the Farnsworth design, and is able to generate neutron flux in the order of one billion neutrons per second. Although the [[Energy conversion efficiency|efficiency]] was very low at first, there were hopes the device could be scaled up, but continued development demonstrated that this approach would be impractical for large machines. Nevertheless, fusion could be achieved using a "lab bench top" type set up for the first time, at minimal cost. This type of fusor found its first application as a portable [[neutron generator]] in the late 1990s. An automated sealed reaction chamber version of this device, commercially named Fusionstar was developed by [[EADS]] but abandoned in 2001. Its successor is the NSD-Fusion [[neutron generator]]. |
|||
=== Magnet quench === |
|||
[[Robert W. Bussard]]'s [[Polywell]] concept is roughly similar to that of the [[Fusor]], but replaces the problematic grid with a magnetically contained electron cloud, which holds the ions in position and provides an accelerating potential. The polywell consists of electromagnet coils arranged in a polyhedral configuration and positively charged to between several tens and low hundreds of kilovolts. This charged magnetic polyhedron is called a MaGrid (Magnetic Grid). Electrons are introduced outside the "quasi-spherical" MaGrid and are accelerated into the MaGrid due to the electric field, similar to a [[magnetic bottle]]. Within the MaGrid, magnetic fields confine most of the electrons and those that escape are retained by the electric field. This configuration traps the electrons in the middle of the device, focusing them near the center to produce a virtual cathode (negative electric potential). The virtual cathode accelerates and confines the ions to be fused which, except for minimal losses, never reach the physical structure of the MaGrid. Bussard had reported a fusion rate of 10<sup>9</sup> per second running D-D fusion reactions at only 12.5 kV (based on [[neutron detection|detecting]] a total of nine neutrons in five tests. Bussard claimed a scaled-up version of 2.5–3 m in diameter, would operated at over 100 MW net power (fusion power scales as the fourth power of the B field and the cube of the size)<ref name="IAC2006">[http://www.askmar.com/ConferenceNotes/2006-9%20IAC%20Paper.pdf "The Advent of Clean Nuclear Fusion: Super-performance Space Power and Propulsion"], Robert W. Bussard, Ph.D., 57th International Astronautical Congress, October 2–6, 2006</ref> |
|||
A [[magnet quench]] is an abnormal termination of magnet operation that occurs when part of the superconducting coil exits the superconducting state (becomes normal). This can occur because the field inside the magnet is too large, the rate of change of field is too large (causing [[eddy current]]s and resultant [[Joule heating|heating]] in the copper support matrix), or a combination of the two. |
|||
More rarely a magnet defect can cause a quench. When this happens, that particular spot is subject to rapid [[Joule heating]] from the current, which raises the [[temperature]] of the surrounding regions. This pushes those regions into the normal state as well, which leads to more heating in a chain reaction. The entire magnet rapidly becomes normal over several seconds, depending on the size of the superconducting coil. This is accompanied by a loud bang as the energy in the magnetic field is converted to heat, and the [[cryogenics|cryogenic]] fluid boils away. The abrupt decrease of current can result in [[kilovolt]] inductive voltage spikes and arcing. Permanent damage to the magnet is rare, but components can be damaged by localized heating, high voltages, or large mechanical forces. |
|||
A recent{{When|date=April 2011}} area of study is the [[magneto-inertial fusion]] (MIF) concept, which combines some form of external inertial compression (like lasers) with further compression through an external magnet (like pinch devices). The magnetic field traps heat within the inertial core, causing a variety of effects that improves fusion rates. These improvements are relatively minor, however the magnetic drivers themselves are inexpensive compared to lasers or other systems. There is hope for a [[sweet spot]] that allows the combination of features from these devices to create low-density but also low-cost fusion devices. A similar concept is the [[magnetized target fusion]] device, which uses a magnetic field in an external metal shell to achieve the same basic goals. |
|||
In practice, magnets usually have safety devices to stop or limit the current when a quench is detected. If a large magnet undergoes a quench, the inert vapor formed by the evaporating cryogenic fluid can present a significant [[asphyxiation]] hazard to operators by displacing breathable air. |
|||
According to [[Eric Lerner]], [[Focus fusion]] takes place in a [[dense plasma focus]]<ref name="PB11">[http://arxiv.org/ftp/arxiv/papers/0710/0710.3149.pdf "ADVANCES TOWARDS PB11 FUSION WITH THE DENSE PLASMA FOCUS"], Eric Lerner, Lawrenceville Plasma Physics, 2008</ref>, which typically consists of two coaxial cylindrical electrodes made from copper or beryllium and housed in a vacuum chamber containing a low-pressure gas, which is used as the reactor fuel. An electrical pulse is applied across the electrodes, producing heating and a magnetic field. The current forms the hot gas into many minuscule vortices perpendicular to the surfaces of the electrodes, which then migrate to the end of the inner electrode to pinch-and-twist off as tiny balls of plasma called plasmoids. The electron beam collides with the plasmoid, heating it to fusion temperatures. This will, in principle, yield more energy in the beams than was input to form them.{{Citation needed|date=February 2010}} |
|||
A large section of the superconducting magnets in [[CERN]]'s [[Large Hadron Collider]] unexpectedly quenched during start-up operations in 2008, destroying multiple magnets.<ref>{{Cite book|url=https://edms.cern.ch/ui/file/973073/1/Report_on_080919_incident_at_LHC__2_.pdf|title=Interim Summary Report on the Analysis of the 19 September 2008 Incident at the LHC|publisher=CERN|year=2008}}</ref> In order to prevent a recurrence, the LHC's superconducting magnets are equipped with fast-ramping heaters that are activated when a quench event is detected. The dipole bending magnets are connected in series. Each power circuit includes 154 individual magnets, and should a quench event occur, the entire combined stored energy of these magnets must be dumped at once. This energy is transferred into massive blocks of metal that heat up to several hundred degrees Celsius—because of resistive heating—in seconds. A magnet quench is a "fairly routine event" during the operation of a particle accelerator.<ref>{{cite web|last=Peterson|first=Tom|title=Explain it in 60 seconds: Magnet Quench|url=http://www.symmetrymagazine.org/article/november-2008/explain-it-in-60-seconds-magnet-quench|website=Symmetry Magazine|date=November 2008 |publisher=[[Fermilab]]/[[SLAC]]|access-date=15 February 2013}}</ref> |
|||
==== Non-power generating approaches==== |
|||
A more subtle technique is to use more unusual particles to catalyse fusion. The best known of these is [[Muon-catalyzed fusion]] which uses muons, which behave somewhat like electrons and replace the electrons around the atoms. These muons allow atoms to get much closer and thus reduce the kinetic energy required to initiate fusion. Muons require more energy to produce than can be obtained from muon-catalysed fusion, making this approach impractical for the generation of power. |
|||
=== Effluents === |
|||
In April 2005, a team from [[UCLA]] [http://www.discover.com/issues/jan-06/features/physics/ announced] it had devised a way of producing fusion using a machine that "fits on a lab bench", using [[lithium tantalate]] to generate enough voltage to smash deuterium atoms together. However, the process does not generate net power. See [[Pyroelectric fusion]]. Such a device would be useful in the same sort of roles as the fusor. |
|||
The natural product of the fusion reaction is a small amount of [[helium]], which is harmless to life. Hazardous tritium is difficult to retain completely. |
|||
Although tritium is volatile and biologically active, the health risk posed by a release is much lower than that of most radioactive contaminants, because of tritium's short half-life (12.32 years) and very low decay energy (~14.95 keV), and because it does not [[bioaccumulation|bioaccumulate]] (it cycles out of the body as water, with a [[biological half-life]] of 7 to 14 days).<ref name="nuclearsafety-petrangeli">{{cite book|first=Gianni |last=Petrangeli|title=Nuclear Safety|url={{google books |plainurl=y |id=5X2Hxad9BoQC|page=430}} |date=2006|publisher=Butterworth-Heinemann|isbn=978-0750667234|page=430}}</ref> ITER incorporates total containment facilities for tritium.<ref name="ITER" /> |
|||
====Abandoned and discredited approaches==== |
|||
Some scientists reported excess heat, neutrons, tritium, helium and other nuclear effects in so-called [[cold fusion]] systems, which for a time gained interest as showing promise. Hopes fell when replication failures were weighed in view of several reasons cold fusion is not likely to occur, the discovery of possible sources of experimental error, and finally the discovery that Fleischmann and Pons had not actually detected nuclear reaction byproducts.<ref>{{harvnb|Browne|1989}}, {{harvnb|Close|1992}}, {{harvnb|Huizenga|1993}}, {{harvnb|Taubes|1993}}</ref> By late 1989, most scientists considered cold fusion claims dead,<ref name="Browne_1989">{{harvnb|Browne|1989}}</ref> and cold fusion subsequently gained a reputation as [[pathological science]].<ref name="nytdoe"> |
|||
{{Cite news |
|||
|author= |
|||
|date=2004-03-25 |
|||
|title=US will give cold fusion a second look |
|||
|url=http://query.nytimes.com/gst/fullpage.html?res=9C01E0DC1530F936A15750C0A9629C8B63 |
|||
|publisher=[[The New York Times]] |
|||
|accessdate=2009-02-08 |
|||
| first=Kenneth |
|||
| last=Chang |
|||
}}</ref> However, a small community of researchers continues to investigate cold fusion<ref name="Browne_1989"/><ref>{{harvnb|Voss|1999}}, {{harvnb|Platt|1998}}, {{harvnb|Goodstein|1994}}, {{harvnb|Van Noorden|2007}}, {{harvnb|Beaudette|2002}}, {{harvnb|Feder|2005}}, {{harvnb|Hutchinson|2006}}, {{harvnb|Kruglinksi|2006}}, {{harvnb|Adam|2005}}</ref><ref name="nytscorn"> |
|||
{{Cite news |
|||
| author = William J. Broad |
|||
| date = 31 October 1989 |
|||
| title = Despite Scorn, Team in Utah Still Seeks Cold-Fusion Clues |
|||
| url = http://query.nytimes.com/gst/fullpage.html?res=950DE6DA1331F932A05753C1A96F948260&pagewanted=all |
|||
| work = [[The New York Times]] |
|||
| pages = C1 |
|||
}}</ref><ref name="wired march 2009">{{harvnb|Randy|2009|ref=CITEREFRandy2009}}</ref> claiming to replicate Fleishmann and Pons' results including nuclear reaction byproducts.<ref name="ACS Press Release"> |
|||
{{cite press |
|||
|url=http://www.eurekalert.org/pub_releases/2009-03/acs-fr031709.php |
|||
|title='Cold fusion' rebirth? New evidence for existence of controversial energy source |
|||
|publisher=[[American Chemical Society]] |
|||
}}</ref><ref name="Hagelstein et al. 2004">{{harvnb|Hagelstein et al.|2004|ref=CITEREFDOE2004}}</ref> Claims related to cold fusion are largely disbelieved in the mainstream scientific community.<ref name="Feder 2005">{{harvnb|Feder|2005}}</ref> In 1989, the majority of a review panel organized by the [[US Department of Energy]] (DOE) found that the evidence for the discovery of a new nuclear process was not persuasive. A second DOE review, convened in 2004 to look at new research, reached conclusions similar to the first.<ref>{{harvnb|Choi|2005}}, {{harvnb|Feder|2005}}, {{harvnb|US DOE|2004|ref=CITEREFDOE2004r}}</ref> |
|||
=== Radioactive waste === |
|||
Research into [[sonoluminescence]] induced fusion, sometimes known as "''[[bubble fusion]]''", also continues, although it is met with as much skepticism as cold fusion is by most of the scientific community. |
|||
{{See also|Radioactive waste}} |
|||
Fusion reactors create far less radioactive material than fission reactors. Further, the material it creates is less damaging biologically, and the radioactivity dissipates within a time period that is well within existing engineering capabilities for safe long-term waste storage.<ref name="demonstration">{{Cite journal |last1=Gonzalez de Vicente |first1=Sehila M. |last2=Smith |first2=Nicholas A. |last3=El-Guebaly |first3=Laila |last4=Ciattaglia |first4=Sergio |last5=Di Pace |first5=Luigi |last6=Gilbert |first6=Mark |last7=Mandoki |first7=Robert |last8=Rosanvallon |first8=Sandrine |last9=Someya |first9=Youji |last10=Tobita |first10=Kenji |last11=Torcy |first11=David |date=2022-08-01 |title=Overview on the management of radioactive waste from fusion facilities: ITER, demonstration machines and power plants |journal=Nuclear Fusion |volume=62 |issue=8 |pages=085001 |doi=10.1088/1741-4326/ac62f7 |bibcode=2022NucFu..62h5001G |s2cid=247920590 |issn=0029-5515|doi-access=free }}</ref> In specific terms, except in the case of [[aneutronic fusion]],<ref>{{Cite book|last1=Harms|first1=A. A.|url=https://books.google.com/books?id=DD0sZgutqowC&pg=PA8|title=Principles of Fusion Energy: An Introduction to Fusion Energy for Students of Science and Engineering|last2=Schoepf|first2=Klaus F.|last3=Kingdon|first3=David Ross|date=2000|publisher=World Scientific|isbn=978-9812380333|language=en}}</ref><ref>{{Cite journal|last1=Carayannis|first1=Elias G.|last2=Draper|first2=John|last3=Iftimie|first3=Ion A.|date=2020|title=Nuclear Fusion Diffusion: Theory, Policy, Practice, and Politics Perspectives |url=https://ieeexplore.ieee.org/document/9078039|journal=IEEE Transactions on Engineering Management|volume=69 |issue=4 |pages=1237–1251|doi=10.1109/TEM.2020.2982101|s2cid=219001461|issn=1558-0040}}</ref> the neutron flux turns the structural materials radioactive. The amount of radioactive material at shut-down may be comparable to that of a fission reactor, with important differences. The half-lives of fusion and neutron activation [[radioisotopes]] tend to be less than those from fission, so that the hazard decreases more rapidly. Whereas fission reactors produce waste that remains radioactive for thousands of years, the radioactive material in a fusion reactor (other than tritium) would be the reactor core itself and most of this would be radioactive for about 50 years, with other low-level waste being radioactive for another 100 years or so thereafter.<ref>{{cite journal |first1=Anil |last1=Markandya |first2=Paul |last2=Wilkinson |s2cid=25504602 |url=http://www.thelancet.com/journals/lancet/article/PIIS0140-6736(07)61253-7/fulltext |journal=The Lancet |volume=370 |issue=9591 |year=2007 |title=Electricity generation and health |pages=979–990 |doi=10.1016/S0140-6736(07)61253-7 |pmid=17876910 |access-date=February 21, 2018}}</ref> The fusion waste's short half-life eliminates the challenge of long-term storage. By 500 years, the material would have the same [[radiotoxicity]] as [[coal ash]].<ref name="WorldEnergyCouncil">{{cite web |last1=Hamacher |first1=T. |last2=Bradshaw |first2=A. M. |date=October 2001 |title=Fusion as a Future Power Source: Recent Achievements and Prospects |url=http://www.worldenergy.org/wec-geis/publications/default/tech_papers/18th_Congress/downloads/ds/ds6/ds6_5.pdf |archive-url=https://web.archive.org/web/20040506065141/http://www.worldenergy.org/wec-geis/publications/default/tech_papers/18th_Congress/downloads/ds/ds6/ds6_5.pdf |archive-date=2004-05-06 |publisher=World Energy Council}}</ref> |
|||
==Key design areas== |
|||
Nonetheless, classification as intermediate level waste rather than low-level waste may complicate safety discussions.<ref>{{Cite journal|last1=Nicholas|first1=T. E. G.|last2=Davis|first2=T. P.|last3=Federici|first3=F.|last4=Leland|first4=J.|last5=Patel|first5=B. S.|last6=Vincent|first6=C.|last7=Ward|first7=S. H.|date=2021-02-01|title=Re-examining the role of nuclear fusion in a renewables-based energy mix|url=https://www.sciencedirect.com/science/article/pii/S0301421520307540|journal=Energy Policy|language=en|volume=149|pages=112043|doi=10.1016/j.enpol.2020.112043|issn=0301-4215|arxiv=2101.05727|bibcode=2021EnPol.14912043N |s2cid=230570595}}</ref><ref name="demonstration" /> |
|||
The choice of materials is less constrained than in conventional fission, where many materials are required for their specific [[neutron cross-section]]s. Fusion reactors can be designed using "low activation", materials that do not easily become radioactive. [[Vanadium]], for example, becomes much less radioactive than [[stainless steel]].<ref>{{Cite journal |last1=Cheng |first1=E. T. |last2=Muroga |first2=Takeo |date=2001 |title=Reuse of Vanadium Alloys in Power Reactors |url=http://dx.doi.org/10.13182/fst01-a11963369 |journal=Fusion Technology |volume=39 |issue=2P2 |pages=981–985 |bibcode=2001FuTec..39..981C |doi=10.13182/fst01-a11963369 |issn=0748-1896 |s2cid=124455585}}</ref> [[Carbon fiber]] materials are also low-activation, are strong and light, and are promising for laser-inertial reactors where a magnetic field is not required.<ref>{{Cite journal|last1=Streckert|first1=H. H.|last2=Schultz|first2=K. R.|last3=Sager|first3=G. T.|last4=Kantncr|first4=R. D.|date=1996-12-01|title=Conceptual Design of Low Activation Target Chamber and Components for the National Ignition Facility|url=https://doi.org/10.13182/FST96-A11962981|journal=Fusion Technology|volume=30|issue=3P2A|pages=448–451|doi=10.13182/FST96-A11962981|bibcode=1996FuTec..30..448S |issn=0748-1896|citeseerx=10.1.1.582.8236}}</ref> |
|||
===Confinement=== |
|||
[[Image:IFE and MFE parameter space.svg|thumb|right|350px|Parameter space occupied by [[inertial fusion energy]] and [[magnetic fusion energy]] devices as of the mid 1990s. The regime allowing thermonuclear ignition with high gain lies near the upper right corner of the plot.]] |
|||
Confinement refers to all the conditions necessary to keep a plasma dense and hot long enough to undergo fusion: |
|||
*[[Mechanical equilibrium|Equilibrium]]: There must be no net forces on any part of the plasma, otherwise it will rapidly disassemble. The exception, of course, is [[inertial confinement fusion|inertial confinement]], where the relevant physics must occur faster than the disassembly time. |
|||
*[[Plasma stability|Stability]]: The plasma must be so constructed that small deviations are restored to the initial state, otherwise some unavoidable disturbance will occur and grow exponentially until the plasma is destroyed. |
|||
*Transport: The loss of particles and heat in all channels must be sufficiently slow. The word "confinement" is often used in the restricted sense of "energy confinement". |
|||
=== Nuclear proliferation === |
|||
The first human-made, large-scale fusion reaction was the test of the [[hydrogen bomb]], [[Ivy Mike]], in 1952. As part of the [[PACER (fusion)|PACER]] project, it was once proposed to use hydrogen bombs as a source of power by detonating them in underground caverns and then generating electricity from the heat produced, but such a power plant is unlikely ever to be constructed, for a variety of reasons.{{Citation needed|date=February 2010}} ''Controlled'' thermonuclear fusion (CTF) refers to the alternative of continuous power production, or at least the use of explosions that are so small that they do not destroy a significant portion of the machine that produces them.{{Citation needed|date=October 2009}} |
|||
{{Main|Nuclear proliferation}} |
|||
In some scenarios, fusion power technology could be adapted to produce materials for military purposes. A huge amount of [[tritium]] could be produced by a fusion power station; tritium is used in the trigger of hydrogen bombs and in modern [[boosted fission weapon]]s, but it can be produced in other ways. The energetic neutrons from a fusion reactor could be used to breed weapons-grade [[plutonium]] or [[uranium]] for an atomic bomb (for example by transmutation of {{chem|238|U}} to {{chem|239|Pu}}, or {{chem|232|Th}} to {{chem|233|U}}). |
|||
To produce self-sustaining fusion, the energy released by the reaction (or at least a fraction of it) must be used to heat new reactant nuclei and keep them hot long enough that they also undergo fusion reactions. Retaining the heat is called energy confinement and may be accomplished in a number of ways.{{Citation needed|date=October 2009}} |
|||
A study conducted in 2011 assessed three scenarios:<ref name="ProliferationRisk_Goldston" /> |
|||
The hydrogen bomb really has no confinement at all. The fuel is simply allowed to fly apart, but it takes a certain length of time to do this, and during this time fusion can occur. This approach is called [[inertial confinement fusion|inertial confinement]]. If more than milligram quantities of fuel are used (and efficiently fused), the explosion would destroy the machine, so theoretically, controlled thermonuclear fusion using inertial confinement would be done using tiny pellets of fuel which explode several times a second. To induce the explosion, the pellet must be compressed to about 30 times solid density with energetic beams. If the beams are focused directly on the pellet, it is called direct drive, which can in principle be very efficient, but in practice it is difficult to obtain the needed uniformity.{{Citation needed|date=October 2009}} An alternative approach is indirect drive, in which the beams heat a shell, and the shell radiates [[x-rays]], which then implode the pellet. The beams are commonly laser beams, but heavy and light [[ion beam]]s and electron beams have all been investigated.{{Citation needed|date=October 2009}} |
|||
* Small-scale fusion station: As a result of much higher power consumption, heat dissipation and a more recognizable design compared to enrichment [[gas centrifuge]]s, this choice would be much easier to detect and therefore implausible.<ref name="ProliferationRisk_Goldston" /> |
|||
* Commercial facility: The production potential is significant. But no fertile or fissile substances necessary for the production of weapon-usable materials needs to be present at a civil fusion system at all. If not shielded, detection of these materials can be done by their characteristic [[Gamma Radiation|gamma radiation]]. The underlying redesign could be detected by regular design information verification. In the (technically more feasible) case of solid breeder blanket modules, it would be necessary for incoming components to be inspected for the presence of fertile material,<ref name="ProliferationRisk_Goldston" /> otherwise plutonium for several weapons could be produced each year.<ref name="StrongNeutronSources" /> |
|||
* Prioritizing weapon-grade material regardless of secrecy: The fastest way to produce weapon-usable material was seen in modifying a civil fusion power station. No weapons-compatible material is required during civil use. Even without the need for covert action, such a modification would take about two months to start production and at least an additional week to generate a significant amount. This was considered to be enough time to detect a military use and to react with diplomatic or military means. To stop the production, a military destruction of parts of the facility while leaving out the reactor would be sufficient.<ref name="ProliferationRisk_Goldston" /> |
|||
Another study concluded "...large fusion reactors—even if not designed for fissile material breeding—could easily produce several hundred kg Pu per year with high weapon quality and very low source material requirements." It was emphasized that the implementation of features for intrinsic proliferation resistance might only be possible at an early phase of research and development.<ref name="StrongNeutronSources" /> The theoretical and computational tools needed for hydrogen bomb design are closely related to those needed for [[inertial confinement fusion]], but have very little in common with magnetic confinement fusion. |
|||
Inertial confinement produces plasmas with impressively high densities and temperatures, and appears to be best suited to weapons research, [[X-ray generation]], very small reactors, and perhaps in the distant future, spaceflight.{{Citation needed|date=October 2009}} They require fuel pellets with close to a perfect shape in order to generate a symmetrical inward [[shock wave]] to produce the high-density plasma, and in practice these have proven difficult to produce. A recent development in the field of laser induced ICF is the use of ultrashort pulse multi-[[petawatt]] lasers to heat the plasma of an imploding pellet at exactly the moment of greatest density after it is imploded conventionally using terawatt scale lasers. This research will be carried out on the (currently being built) OMEGA EP petawatt and [[LLE|OMEGA]] lasers at the [[University of Rochester]] and at the GEKKO XII laser at the institute for laser engineering in Osaka Japan, which if fruitful, may have the effect of greatly reducing the cost of a laser fusion based power source.{{Citation needed|date=October 2009}} |
|||
=== Fuel reserves === |
|||
At the temperatures required for fusion, the fuel is in the form of a plasma with very good [[electrical conductivity]]. This opens the possibility to confine the fuel and the energy with [[magnetic field]]s, an idea known as [[magnetic fusion energy|magnetic confinement]]. The [[Lorenz force]] works only perpendicular to the magnetic field, so that the first problem is how to prevent the plasma from leaking out the ends of the field lines. There are basically two solutions.{{Citation needed|date=October 2009}} |
|||
Fusion power commonly proposes the use of deuterium as fuel and many current designs also use [[lithium]]. Assuming a fusion energy output equal to the 1995 global power output of about 100 [[exa-|E]]J/yr (= 1 × 10<sup>20</sup> J/yr) and that this does not increase in the future, which is unlikely, then known current lithium reserves would last 3000 years. Lithium from sea water would last 60 million years, however, and a more complicated fusion process using only deuterium would have fuel for 150 billion years.<ref>{{cite web|url=http://www.agci.org/dB/PDFs/03S2_MMauel_SafeFusion%3F.pdf |archive-url=https://web.archive.org/web/20110727135814/http://www.agci.org/dB/PDFs/03S2_MMauel_SafeFusion?.pdf |url-status=dead |archive-date=2011-07-27 |title=Energy for Future Centuries |access-date=2013-06-22 }}</ref> To put this in context, 150 billion years is close to 30 times the remaining lifespan of the Sun,<ref name="sunlife">{{cite web |last=Christian |first=Eric |display-authors=etal |title=Cosmicopia |url=http://helios.gsfc.nasa.gov/qa_sun.html#sunlife |url-status=dead |archive-url=https://web.archive.org/web/20111106095009/http://helios.gsfc.nasa.gov/qa_sun.html#sunlife |archive-date=November 6, 2011 |access-date=2009-03-20 |publisher=NASA}}</ref> and more than 10 times the estimated age of the universe. |
|||
== Economics == |
|||
The first is to use the [[magnetic mirror]] effect. If particles following a field line encounter a region of higher field strength, then some of the particles will be stopped and reflected. Advantages of a magnetic mirror power plant would be simplified construction and maintenance due to a linear topology and the potential to apply direct conversion in a natural way, but the confinement achieved in the experiments was so poor that this approach has been essentially abandoned.{{Citation needed|date=October 2009}} |
|||
The EU spent almost {{nowrap|€10 billion}} through the 1990s.<ref>{{cite web|author=Fusion For Energy|title=Fusion For Energy – Bringing the power of the sun to earth|url=http://www.f4e.europa.eu|url-status=dead|archive-url=https://web.archive.org/web/20191129201922/https://f4e.europa.eu/|archive-date=November 29, 2019|access-date=July 17, 2020|website=f4e.europa.eu}}</ref> [[ITER]] represents an investment of over twenty billion dollars, and possibly tens of billions more, including [[In kind|in kind contributions]].<ref>{{Cite journal|date=2016|title=ITER governing council pushes schedule back five years and trims budget|journal=Physics Today|doi=10.1063/pt.5.029905|issn=1945-0699}}</ref><ref>{{Cite journal|year=2018|title=ITER disputes DOE's cost estimate of fusion project|journal=Physics Today|doi=10.1063/PT.6.2.20180416a}}</ref> Under the European Union's [[Framework Programmes for Research and Technological Development|Sixth Framework Programme]], nuclear fusion research received {{nowrap|€750 million}} (in addition to ITER funding), compared with {{nowrap|€810 million}} for sustainable energy research,<ref>{{cite web |title=The Sixth Framework Programme in brief |publisher=ec.europa.eu |url= http://ec.europa.eu/research/fp6/pdf/fp6-in-brief_en.pdf |access-date=30 October 2014}}</ref> putting research into fusion power well ahead of that of any single rival technology. The [[United States Department of Energy]] has allocated $US367M–$US671M every year since 2010, peaking in 2020,<ref>{{cite web |last1=Margraf |first1=Rachel |title=A Brief History of U.S. Funding of Fusion Energy |url=http://large.stanford.edu/courses/2021/ph241/margraf1/ |access-date=21 July 2021}}</ref> with plans to reduce investment to $US425M in its FY2021 Budget Request.<ref>DOE/CF-0167 – Department of Energy FY 2021 Congressional Budget Request, Budget in Brief, February 2020. https://www.energy.gov/sites/default/files/2020/02/f72/doe-fy2021-budget-in-brief_0.pdf {{Webarchive|url=https://web.archive.org/web/20210718212001/https://www.energy.gov/sites/default/files/2020/02/f72/doe-fy2021-budget-in-brief_0.pdf |date=2021-07-18 }}</ref> About a quarter of this budget is directed to support ITER. |
|||
The size of the investments and time lines meant that fusion research was traditionally almost exclusively publicly funded. However, starting in the 2010s, the promise of commercializing a paradigm-changing [[low-carbon energy]] source began to attract a raft of companies and investors.<ref>{{Cite book|editor=Nuttall, William J. |title=Commercialising fusion energy : how small businesses are transforming big science|date=2020|publisher=Institute of Physics |isbn=978-0750327176|oclc=1230513895}}</ref> Over two dozen start-up companies attracted over one billion dollars from roughly 2000 to 2020, mainly from 2015, and a further three billion in funding and milestone related commitments in 2021,<ref>{{Cite book|last=Fusion Energy Sciences Advisory Committee|url=https://usfusionandplasmas.org/wp-content/themes/FESAC/FESAC_Report_2020_Powering_the_Future.pdf|title=Powering the Future: Fusion & Plasmas|publisher=Department of Energy Fusion Energy Sciences|year=2021|location=Washington|pages=ii|language=en}}</ref><ref>{{Cite web|last=Helman|first=Christopher|title=Fueled By Billionaire Dollars, Nuclear Fusion Enters A New Age|url=https://www.forbes.com/sites/christopherhelman/2022/01/02/fueled-by-billionaire-dollars-nuclear-fusion-enters-a-new-age/|access-date=2022-01-14|website=Forbes|language=en}}</ref> with investors including [[Jeff Bezos]], [[Peter Thiel]] and [[Bill Gates]], as well as institutional investors including [[Legal & General]], and energy companies including [[Equinor]], [[Eni]], [[Chevron Corporation|Chevron]],<ref name="Space Race">{{Cite web|last=Windridge|first=Melanie|title=The New Space Race Is Fusion Energy|url=https://www.forbes.com/sites/melaniewindridge/2020/10/07/the-new-space-race-is-fusion-energy/|access-date=2020-10-10|website=Forbes|language=en}}</ref> and the Chinese [[ENN Group]].<ref>{{Citation |last1=Pearson |first1=Richard J. |title=Review of approaches to fusion energy |date=2020 |url=http://dx.doi.org/10.1088/978-0-7503-2719-0ch2 |work=Commercialising Fusion Energy |access-date=2021-12-13 |publisher=IOP Publishing |doi=10.1088/978-0-7503-2719-0ch2 |isbn=978-0750327190 |s2cid=234561187 |last2=Takeda |first2=Shutaro}}</ref><ref>{{Citation |last1=Pearson |first1=Richard J. |title=Pioneers of commercial fusion |date=2020 |url=http://dx.doi.org/10.1088/978-0-7503-2719-0ch7 |work=Commercialising Fusion Energy |access-date=2021-12-13 |publisher=IOP Publishing |doi=10.1088/978-0-7503-2719-0ch7 |isbn=978-0750327190 |s2cid=234528929 |last2=Nuttall |first2=William J.}}</ref><ref name="Diffusion" /> In 2021, Commonwealth Fusion Systems (CFS) obtained $1.8 billion in scale-up funding, and Helion Energy obtained a half-billion dollars with an additional $1.7 billion contingent on meeting milestones.<ref name="Sets Sights">{{Cite web |title=White House Sets Sights on Commercial Fusion Energy |url=https://www.aip.org/fyi/2022/white-house-sets-sights-commercial-fusion-energy |access-date=2022-05-03 |website=www.aip.org |date=April 25, 2022 |language=en}}</ref> |
|||
The second possibility to prevent end losses is to bend the field lines back on themselves, either in circles or more commonly in nested [[torus|toroidal]] surfaces. The most highly developed system of this type is the ''[[tokamak]]'', with the ''[[stellarator]]'' being next most advanced, followed by the [[Reversed field pinch]]. Compact toroids, especially the ''[[Field-Reversed Configuration]]'' and the [[spheromak]], attempt to combine the advantages of toroidal magnetic surfaces with those of a [[simply connected space|simply connected]] (non-toroidal) machine, resulting in a mechanically simpler and smaller confinement area. Compact toroids still have some enthusiastic supporters but are not backed as readily by the majority of the fusion community.{{Citation needed|date=October 2009}} |
|||
Scenarios developed in the 2000s and early 2010s discussed the effects of the commercialization of fusion power on the future of human civilization.<ref name="Giant Step">{{cite web |last1=Lee |first1=Sing |last2=Saw |first2=Sor Heoh |title=Nuclear Fusion Energy – Mankind's Giant Step Forward |url=http://www.plasmafocus.net/IPFS/2010%20Papers/LSmankind.pdf |access-date=30 October 2014 |publisher=HPlasmafocus.net}}</ref> Using nuclear fission as a guide, these saw ITER and later [[DEMOnstration Power Station|DEMO]] as bringing online the first commercial reactors around 2050 and a rapid expansion after mid-century.<ref name="Giant Step" /> Some scenarios emphasized "fusion nuclear science facilities" as a step beyond ITER.<ref>{{Cite journal|last1=Kessel|first1=C. E.|last2=Blanchard|first2=J. P.|last3=Davis|first3=A.|last4=El-Guebaly|first4=L.|last5=Ghoniem|first5=N.|last6=Humrickhouse|first6=P. W.|last7=Malang|first7=S.|last8=Merrill|first8=B. J.|last9=Morley|first9=N. B.|last10=Neilson|first10=G. H.|last11=Rensink|first11=M. E.|date=2015-09-01|title=The Fusion Nuclear Science Facility, the Critical Step in the Pathway to Fusion Energy|url=https://doi.org/10.13182/FST14-953|journal=Fusion Science and Technology|volume=68|issue=2|pages=225–236|doi=10.13182/FST14-953|bibcode=2015FuST...68..225K |osti=1811772|s2cid=117842168|issn=1536-1055}}</ref><ref name="pilot plants">{{Cite journal |last1=Menard |first1=J. E. |last2=Brown |first2=T. |last3=El-Guebaly |first3=L. |last4=Boyer |first4=M. |last5=Canik |first5=J. |last6=Colling |first6=B. |last7=Raman |first7=R. |last8=Wang |first8=Z. |last9=Zhai |first9=Y. |last10=Buxton |first10=P. |last11=Covele |first11=B. |date=2016-10-01 |title=Fusion nuclear science facilities and pilot plants based on the spherical tokamak |url=https://iopscience.iop.org/article/10.1088/0029-5515/56/10/106023 |journal=Nuclear Fusion |volume=56 |issue=10 |pages=106023 |bibcode=2016NucFu..56j6023M |doi=10.1088/0029-5515/56/10/106023 |issn=0029-5515 |s2cid=125184562}}</ref> However, the economic obstacles to tokamak-based fusion power remain immense, requiring investment to fund prototype tokamak reactors<ref>{{Cite journal|last=Cardozo|first=N. J. Lopes|date=2019-02-04|title=Economic aspects of the deployment of fusion energy: the valley of death and the innovation cycle|journal=Philosophical Transactions of the Royal Society A: Mathematical, Physical and Engineering Sciences |volume=377|issue=2141|pages=20170444|doi=10.1098/rsta.2017.0444|pmid=30967058|bibcode=2019RSPTA.37770444C|s2cid=106411210|issn=1364-503X|doi-access=free}}</ref> and development of new supply chains,<ref>{{Cite journal|last=Surrey|first=E.|date=2019-02-04|title=Engineering challenges for accelerated fusion demonstrators|journal=Philosophical Transactions of the Royal Society A: Mathematical, Physical and Engineering Sciences|volume=377|issue=2141|pages=20170442|doi=10.1098/rsta.2017.0442|pmid=30967054|pmc=6365852|bibcode=2019RSPTA.37770442S|issn=1364-503X|doi-access=free}}</ref> a problem which will affect any kind of fusion reactor.<ref>{{Cite book |url=https://drive.google.com/file/d/1rXrzFQ83DL2q68UB3BjU2jdFVSRHxxBF/view |title=The Fusion Industry Supply Chain: Opportunities and Challenges |publisher=Fusion Industry Association |year=2023 |location=Washington, DC}}</ref> Tokamak designs appear to be labour-intensive,<ref>{{Cite journal|last1=Banacloche|first1=Santacruz|last2=Gamarra|first2=Ana R.|last3=Lechon|first3=Yolanda|last4=Bustreo|first4=Chiara|date=2020-10-15|title=Socioeconomic and environmental impacts of bringing the sun to earth: A sustainability analysis of a fusion power plant deployment |url=https://www.sciencedirect.com/science/article/pii/S0360544220315681|journal=Energy|language=en|volume=209|pages=118460|doi=10.1016/j.energy.2020.118460|bibcode=2020Ene...20918460B |s2cid=224952718|issn=0360-5442}}</ref> while the commercialization risk of alternatives like inertial fusion energy is high due to the lack of government resources.<ref>{{Cite journal|last=Koepke|first=M. E.|date=2021-01-25|title=Factors influencing the commercialization of inertial fusion energy|url= |journal=Philosophical Transactions of the Royal Society A: Mathematical, Physical and Engineering Sciences|language=en|volume=379|issue=2189|pages=20200020|doi=10.1098/rsta.2020.0020|issn=1364-503X|pmc=7741007|pmid=33280558|bibcode=2021RSPTA.37900020K}}</ref> |
|||
Finally, there are also ''[[Inertial electrostatic confinement|electrostatic confinement fusion]]'' systems, in which [[ion]]s in the reaction chamber are confined and held at the center of the device by electrostatic forces, as in the [[Farnsworth-Hirsch Fusor]], which is not believed to be able to be developed into a power plant. The [[Polywell]], an advanced variant of the [[fusor]],{{Citation needed|date=October 2009}} has shown a degree of research interest as of late; however, the technology is relatively immature,{{Citation needed|date=October 2009}} and major scientific and engineering questions remain which researchers under the auspices of the U.S. [[Office of Naval Research]] hope to further investigate.{{Citation needed|date=October 2009}} |
|||
Scenarios since 2010 note computing and material science advances enabling multi-phase national or cost-sharing "Fusion Pilot Plants" (FPPs) along various technology pathways,<ref>{{Cite journal |last1=Menard |first1=J. E. |last2=Bromberg |first2=L. |last3=Brown |first3=T. |last4=Burgess |first4=T. |last5=Dix |first5=D. |last6=El-Guebaly |first6=L. |last7=Gerrity |first7=T. |last8=Goldston |first8=R. J. |last9=Hawryluk |first9=R. J. |last10=Kastner |first10=R. |last11=Kessel |first11=C. |date=2011-10-01 |title=Prospects for pilot plants based on the tokamak, spherical tokamak and stellarator |url=https://iopscience.iop.org/article/10.1088/0029-5515/51/10/103014 |journal=Nuclear Fusion |volume=51 |issue=10 |pages=103014 |bibcode=2011NucFu..51j3014M |doi=10.1088/0029-5515/51/10/103014 |issn=0029-5515 |s2cid=55781189}}</ref><ref name="pilot plants" /><ref name="Tokamak">{{Cite journal|last1=Hiwatari|first1=Ryoji|last2=Goto |first2=Takuya|date=2019-03-19|title=Assessment on Tokamak Fusion Power Plant to Contribute to Global Climate Stabilization in the Framework of Paris Agreement|journal=Plasma and Fusion Research |volume=14|pages=1305047|bibcode=2019PFR....1405047H|doi=10.1585/pfr.14.1305047|issn=1880-6821|doi-access=free}}</ref><ref>{{Cite book|last=((National Academies of Sciences, Engineering, and Medicine (U.S.). Committee on a Strategic Plan for U.S. Burning Plasma Research))|title=Final report of the Committee on a Strategic Plan for U.S. Burning Plasma Research|isbn=978-0309487443|location=Washington, DC|oclc=1104084761}}</ref><ref>{{Cite book|url=https://drive.google.com/file/d/1w0TKL_Jn0tKUBgUc8RC1s5fIOViH5pRK/view|title=A Community Plan for Fusion Energy and Discovery Plasma Sciences|publisher=American Physical Society Division of Plasma Physics Community Planning Process|year=2020|location=Washington, DC}}</ref><ref>{{Cite web|date=2020-04-07|title=US Plasma Science Strategic Planning Reaches Pivotal Phase|url=https://www.aip.org/fyi/2020/us-plasma-science-strategic-planning-reaches-pivotal-phase|access-date=2020-10-08|website=www.aip.org|language=en}}</ref> such as the UK [[Spherical Tokamak for Energy Production]], within the 2030–2040 time frame.<ref>{{Cite news|last1=Asmundssom|first1=Jon|last2=Wade|first2=Will|title=Nuclear Fusion Could Rescue the Planet from Climate Catastrophe|work=Bloomberg|url=https://www.bloomberg.com/news/features/2019-09-28/startups-take-aim-at-nuclear-fusion-energy-s-biggest-challenge|access-date=2020-09-21}}</ref><ref>{{Cite news|last=Michaels|first=Daniel|date=2020-02-06|title=Fusion Startups Step In to Realize Decades-Old Clean Power Dream|language=en-US|work=[[The Wall Street Journal]]|url=https://www.wsj.com/articles/fusion-startups-step-in-to-realize-decades-old-clean-power-dream-11581001383|access-date=2020-10-08|issn=0099-9660}}</ref><ref name="Early Markets">{{Cite journal|last1=Handley|first1=Malcolm C.|last2=Slesinski|first2=Daniel|last3=Hsu|first3=Scott C.|date=2021-07-10|title=Potential Early Markets for Fusion Energy|url=http://dx.doi.org/10.1007/s10894-021-00306-4|journal=Journal of Fusion Energy|volume=40|issue=2|page=18|doi=10.1007/s10894-021-00306-4|issn=0164-0313|arxiv=2101.09150|s2cid=231693147}}</ref> Notably, in June 2021, General Fusion announced it would accept the UK government's offer to host the world's first substantial [[Public–private partnership|public-private partnership]] fusion demonstration plant, at [[Culham Centre for Fusion Energy]].<ref>{{Cite journal|last=Ball|first=Philip|date=2021-11-17|title=The chase for fusion energy|journal=Nature|language=en|volume=599|issue=7885|pages=352–366|doi=10.1038/d41586-021-03401-w|pmid=34789909|s2cid=244346561|doi-access=free}}</ref> The plant will be constructed from 2022 to 2025 and is intended to lead the way for commercial pilot plants in the late 2025s. The plant will be 70% of full scale and is expected to attain a stable plasma of 150 million degrees.<ref>{{Cite web|date=2021-06-16|title=A Historic Decision: To Demonstrate Practical Fusion at Culham|url=https://generalfusion.com/2021/06/a-historic-decision-to-demonstrate-practical-fusion-at-culham/|access-date=2021-06-18|website=General Fusion|language=en-US}}</ref> In the United States, cost-sharing public-private partnership FPPs appear likely,<ref>{{Cite web|last=Holland|first=Andrew|date=2021-07-15|title=Congress Would Fund Fusion Cost-Share Program in Committee-Passed Appropriations Bill|url=https://www.fusionindustryassociation.org/post/house-energy-water-subcommittee-includes-cost-share-program-in-their-passed-appropriation-bill|access-date=2021-07-16|website=Fusion Industry Assn|language=en|archive-date=2023-04-20 |archive-url=https://web.archive.org/web/20230420002948/https://www.fusionindustryassociation.org/post/house-energy-water-subcommittee-includes-cost-share-program-in-their-passed-appropriation-bill|url-status=dead}}</ref> and in 2022 the DOE announced a new Milestone-Based Fusion Development Program as the centerpiece of its Bold Decadal Vision for Commercial Fusion Energy,<ref>{{Cite web |last=Sailer |first=Sandy |date=2023-05-31 |title=Department of Energy Announces Milestone Public-Private Partnership Awards |url=https://www.fusionindustryassociation.org/department-of-energy-announces-milestone-public-private-partnership-awards/ |access-date=2023-06-01 |website=Fusion Industry Association |language=en-US}}</ref> which envisages private sector-led teams delivering FPP pre-conceptual designs, defining technology roadmaps, and pursuing the R&D necessary to resolve critical-path scientific and technical issues towards an FPP design.<ref>{{Cite journal |last=Hsu |first=Scott C. |date=2023-05-05 |title=U.S. Fusion Energy Development via Public-Private Partnerships |journal=Journal of Fusion Energy |volume=42 |issue=1 |doi=10.1007/s10894-023-00357-9 |s2cid=258489130 |issn=0164-0313|doi-access=free }}</ref> Compact reactor technology based on such demonstration plants may enable commercialization via a fleet approach from the 2030s<ref>{{Cite journal|last1=Spangher|first1=Lucas|last2=Vitter|first2=J. Scott|last3=Umstattd|first3=Ryan|date=2019|title=Characterizing fusion market entry via an agent-based power plant fleet model|journal=Energy Strategy Reviews|volume=26|pages=100404|doi=10.1016/j.esr.2019.100404|issn=2211-467X|doi-access=free|bibcode=2019EneSR..2600404S }}</ref> if early markets can be located.<ref name="Early Markets" /> |
|||
===Materials=== |
|||
{{Main|International Fusion Materials Irradiation Facility}} |
|||
Developing materials for fusion reactors has long been recognized as a problem nearly as difficult and important as that of plasma confinement, but it has received only a fraction of the attention. The neutron flux in a fusion reactor is expected to be about 100 times that in existing [[pressurized water reactor]]s (PWR). Each atom in the blanket of a fusion reactor is expected to be hit by a neutron and displaced about a hundred times before the material is replaced. Furthermore the high-energy neutrons will produce hydrogen and helium in various nuclear reactions that tends to form bubbles at grain boundaries and result in swelling, blistering or embrittlement. One also wishes to choose materials whose primary components and impurities do not result in long-lived radioactive wastes. Finally, the mechanical forces and temperatures are large, and there may be frequent cycling of both. |
|||
The widespread adoption of non-nuclear renewable energy has transformed the energy landscape. Such renewables are projected to supply 74% of global energy by 2050.<ref>{{Cite web|title=Global Energy Perspectives 2019|url=https://www.mckinsey.com/~/media/mckinsey/industries/oil%20and%20gas/our%20insights/global%20energy%20perspective%202019/mckinsey-energy-insights-global-energy-perspective-2019_reference-case-summary.ashx|website=Energy Insights- Mckinsey}}</ref> The steady fall of renewable energy prices challenges the economic competitiveness of fusion power.<ref name="Energy Mix">{{Cite journal|last1=Nicholas|first1=T. E. G.|last2=Davis|first2=T. P.|last3=Federici|first3=F.|last4=Leland|first4=J. E.|last5=Patel|first5=B. S.|last6=Vincent|first6=C.|last7=Ward|first7=S. H.|date=February 2021|title=Re-examining the Role of Nuclear Fusion in a Renewables-Based Energy Mix|journal=Energy Policy|volume=149|pages=112043|arxiv=2101.05727|doi=10.1016/j.enpol.2020.112043|bibcode=2021EnPol.14912043N |s2cid=230570595}}</ref> |
|||
The problem is exacerbated because realistic material tests must expose samples to neutron fluxes of a similar level for a similar length of time as those expected in a fusion power plant. Such a neutron source is nearly as complicated and expensive as a fusion reactor itself would be. Proper materials testing will not be possible in [[ITER]], and a proposed materials testing facility, [[IFMIF]], was still at the design stage in 2005. |
|||
[[File:20201019 Levelized Cost of Energy (LCOE, Lazard) - renewable energy.svg|thumb|upright=1.5|Levelized cost of energy (LCOE) for various sources of energy including wind, solar and nuclear energy<ref name=LazardLCOE_20230412>{{cite web |title=2023 Levelized Cost Of Energy+ |url=https://www.lazard.com/research-insights/2023-levelized-cost-of-energyplus/ |publisher=Lazard |archive-url=https://web.archive.org/web/20230827132200/https://www.lazard.com/research-insights/2023-levelized-cost-of-energyplus/ |archive-date=27 August 2023 |page=9 |date=12 April 2023 |url-status=live}} (Download link labeled "Lazard's LCOE+ (April 2023) (1) PDF—1MB")</ref>]] |
|||
The material of the plasma facing components (PFC) is a special problem. The PFC do not have to withstand large mechanical loads, so neutron damage is much less of an issue. They do have to withstand extremely large thermal loads, up to 10 MW/m², which is a difficult but solvable problem. Regardless of the material chosen, the heat flux can only be accommodated without melting if the distance from the front surface to the coolant is not more than a centimeter or two. The primary issue is the interaction with the plasma. One can choose either a low-[[Atomic number|Z]] material, typified by [[graphite]] although for some purposes [[beryllium]] might be chosen, or a high-[[Atomic number|Z]] material, usually [[tungsten]] with [[molybdenum]] as a second choice. Use of liquid metals (lithium, gallium, tin) has also been proposed, e.g., by injection of 1–5 mm thick streams flowing at 10 m/s on solid substrates. |
|||
Some economists suggest fusion power is unlikely to match other [[renewable energy]] costs.<ref name="Energy Mix" /> Fusion plants are expected to face large start up and [[capital cost]]s. Moreover, operation and maintenance are likely to be costly.<ref name="Energy Mix" /> While the costs of the [[China Fusion Engineering Test Reactor]] are not well known, an EU DEMO fusion concept was projected to feature a [[levelized cost of energy]] (LCOE) of $121/MWh.<ref>{{Cite journal|date=2018-06-01|title=Approximation of the economy of fusion energy|journal=Energy|language=en|volume=152|pages=489–497|doi=10.1016/j.energy.2018.03.130|issn=0360-5442|doi-access=free|last1=Entler|first1=Slavomir|last2=Horacek|first2=Jan|last3=Dlouhy|first3=Tomas|last4=Dostal|first4=Vaclav|bibcode=2018Ene...152..489E }}</ref> |
|||
If graphite is used, the gross erosion rates due to physical and chemical [[sputtering]] would be many meters per year, so one must rely on redeposition of the sputtered material. The location of the redeposition will not exactly coincide with the location of the sputtering, so one is still left with erosion rates that may be prohibitive. An even larger problem is the tritium co-deposited with the redeposited graphite. The tritium inventory in graphite layers and dust in a reactor could quickly build up to many kilograms, representing a waste of resources and a serious radiological hazard in case of an accident. The consensus of the fusion community seems to be that graphite, although a very attractive material for fusion experiments, cannot be the primary PFC material in a commercial reactor. |
|||
Fuel costs are low, but economists suggest that the energy cost for a one-gigawatt plant would increase by $16.5 per MWh for every $1 billion increase in the capital investment in construction. There is also the risk that easily obtained lithium will be used up making batteries. Obtaining it from seawater would be very costly and might require more energy than the energy that would be generated.<ref name="Energy Mix" /> |
|||
The sputtering rate of tungsten can be orders of magnitude smaller than that of carbon, and tritium is not so easily incorporated into redeposited tungsten, making this a more attractive choice. On the other hand, tungsten impurities in a plasma are much more damaging than carbon impurities, and self-sputtering of tungsten can be high, so it will be necessary to ensure that the plasma in contact with the tungsten is not too hot (a few tens of eV rather than hundreds of eV). Tungsten also has disadvantages in terms of eddy currents and melting in off-normal events, as well as some radiological issues. |
|||
In contrast, [[Renewable energy|renewable]] levelized cost of energy estimates are substantially lower. For instance, the 2019 levelized cost of energy of [[solar energy]] was estimated to be $40-$46/MWh, [[Onshore wind farm|on shore wind]] was estimated at $29-$56/MWh, and [[Offshore wind power|offshore wind]] was approximately $92/MWh.<ref>{{Cite web|title=Levelized Cost of Energy and Levelized Cost of Storage 2019|url=http://www.lazard.com/perspective/levelized-cost-of-energy-and-levelized-cost-of-storage-2019/|access-date=2021-06-01|website=Lazard.com|language=en|archive-date=2023-02-19 |archive-url=https://web.archive.org/web/20230219103421/http://www.lazard.com/perspective/levelized-cost-of-energy-and-levelized-cost-of-storage-2019/|url-status=dead}}</ref> |
|||
===Subsystems=== |
|||
In fusion research, achieving a [[fusion energy gain factor]] ''Q'' = 1 is called breakeven and is considered a significant although somewhat artificial milestone. Ignition refers to an infinite ''Q'', that is, a self-sustaining plasma where the losses are made up for by fusion power without any external input. In a practical fusion reactor, some external power will always be required for things like current drive, refueling, profile control, and burn control. A value on the order of ''Q'' = 20 will be required if the plant is to deliver much more energy than it uses internally. |
|||
However, fusion power may still have a role filling energy gaps left by renewables,<ref name="Early Markets" /><ref name="Energy Mix" /> depending on how administration priorities for energy and environmental justice influence the market.<ref name="Sets Sights" /> In the 2020s, socioeconomic studies of fusion that began to consider these factors emerged,<ref>{{Cite journal |last1=Griffiths |first1=Thomas |last2=Pearson |first2=Richard |last3=Bluck |first3=Michael |last4=Takeda |first4=Shutaro |date=2022-10-01 |title=The commercialisation of fusion for the energy market: a review of socio-economic studies |journal=Progress in Energy |volume=4 |issue=4 |pages=042008 |doi=10.1088/2516-1083/ac84bf |bibcode=2022PrEne...4d2008G |s2cid=251145811 |issn=2516-1083|doi-access=free }}</ref> and in 2022 EUROFusion launched its Socio-Economic Studies and Prospective Research and Development strands to investigate how such factors might affect commercialization pathways and timetables.<ref>{{Cite journal |last1=Kembleton |first1=R. |last2=Bustreo |first2=C. |date=2022 |title=Prospective research and development for fusion commercialisation |journal=Fusion Engineering and Design |volume=178 |pages=113069 |doi=10.1016/j.fusengdes.2022.113069 |s2cid=247338079 |issn=0920-3796|doi-access=free |bibcode=2022FusED.17813069K }}</ref> Similarly, in April 2023 Japan announced a national strategy to industrialise fusion.<ref>{{Cite web |last=Otake |first=Tomoko |date=2023-04-14 |title=Japan adopts national strategy on nuclear fusion as competition intensifies |url=https://www.japantimes.co.jp/news/2023/04/14/national/japan-national-strategy-nuclear-fusion/ |access-date=2023-04-19 |website=The Japan Times |language=en-US}}</ref> Thus, fusion power may work in tandem with other renewable energy sources rather than becoming the primary energy source.<ref name="Energy Mix" /> In some applications, fusion power could provide the base load, especially if including integrated thermal storage and cogeneration and considering the potential for retrofitting coal plants.<ref name="Early Markets" /><ref name="Energy Mix" /> |
|||
Despite many differences between possible designs of power plant, there are several systems that are common to most. A fusion power plant, like a [[nuclear power|fission power plant]], is customarily divided into the nuclear island and the balance of plant. The balance of plant converts heat into electricity via [[steam turbine]]s; it is a conventional design area and in principle similar to any other power station that relies on heat generation, whether fusion, fission or [[fossil fuel]] based. |
|||
== Regulation == |
|||
The nuclear island has a plasma chamber with an associated vacuum system, surrounded by plasma-facing components (first wall and divertor) maintaining the vacuum boundary and absorbing the thermal radiation coming from the plasma, surrounded in turn by a [[blanket (disambiguation)|blanket]] where the neutrons are absorbed to breed tritium and heat a working fluid that transfers the power to the balance of plant. If magnetic confinement is used, a magnet system, using primarily cryogenic superconducting magnets, is needed, and usually systems for heating and refueling the plasma and for driving current. In inertial confinement, a driver (laser or accelerator) and a focusing system are needed, as well as a means for forming and positioning the pellets. |
|||
As fusion pilot plants move within reach, legal and regulatory issues must be addressed.<ref>{{Cite journal|last=Holland|first=Andrew|date=2020-11-13|title=Political and commercial prospects for inertial fusion energy|journal=Philosophical Transactions of the Royal Society A: Mathematical, Physical and Engineering Sciences |volume=378|issue=2184|pages=20200008|doi=10.1098/rsta.2020.0008|pmid=33040662|bibcode=2020RSPTA.37800008H|s2cid=222277887|doi-access=free}}</ref> In September 2020, the United States [[National Academy of Sciences]] consulted with private fusion companies to consider a national pilot plant. The following month, the United States Department of Energy, the [[Nuclear Regulatory Commission]] (NRC) and the [[Fusion Industry Association]] co-hosted a public forum to begin the process.<ref name="Space Race" /> In November 2020, the [[International Atomic Energy Agency]] (IAEA) began working with various nations to create safety standards<ref name="Safety in Fusion">{{Cite web|date=2021-05-28|title=Safety in Fusion|url=https://www.iaea.org/fusion-energy/safety-in-fusion|access-date=2021-06-01|website=www.iaea.org|language=en}}</ref> such as dose regulations and [[radioactive waste]] handling.<ref name="Safety in Fusion" /> In January and March 2021, NRC hosted two public meetings on regulatory frameworks.<ref>{{Cite web|last=Slesinski|first=Daniel|date=2021-01-28|title=NRC Hosts Virtual Public Meeting on Developing Options for a Regulatory Framework for Fusion Energy|url=https://www.fusionindustryassociation.org/post/nrc-hosts-virtual-public-meeting-on-developing-options-for-a-regulatory-framework-for-fusion-energy|access-date=2021-02-14|website=Fusion Industry Assn|language=en}}</ref><ref>{{Cite web|last=Slesinski|first=Daniel|date=2021-03-30|title=NRC Hosts Second Virtual Public Meeting on Developing a Regulatory Framework for Fusion Energy|url=https://www.fusionindustryassociation.org/post/nrc-hosts-second-virtual-public-meeting-on-developing-a-regulatory-framework-for-fusion|access-date=2021-04-10|website=Fusion Industry Assn|language=en}}</ref> A public-private cost-sharing approach was endorsed in the 27 December H.R.133 Consolidated Appropriations Act, 2021, which authorized $325 million over five years for a partnership program to build fusion demonstration facilities, with a 100% match from private industry.<ref>{{Cite web|last=Holland|first=Andrew|date=2021-01-05|title=Fusion Legislation Signed into Law|url=https://www.fusionindustryassociation.org/post/fusion-legislation-signed-into-law|access-date=2021-02-14|website=Fusion Industry Assn|language=en}}</ref> |
|||
Subsequently, the UK Regulatory Horizons Council published a report calling for a fusion regulatory framework by early 2022<ref>{{Cite web|last=Windridge|first=Melanie|title=U.K Serious About Fusion: New Report On Regulation Recommends Proportionate, Agile Approach|url=https://www.forbes.com/sites/melaniewindridge/2021/06/02/uk-serious-about-fusion-new-report-on-regulation-recommends-proportionate-agile-approach/|access-date=2021-06-03|website=Forbes|language=en}}</ref> in order to position the UK as a global leader in commercializing fusion power.<ref>{{Cite web|last=Holland|first=Andrew|date=2021-06-01|title=UK Regulatory Horizons Council Issues Report on Fusion Energy Regulation|url=https://www.fusionindustryassociation.org/post/uk-s-regulatory-horizons-council-issues-report-on-fusion-energy-regulation|access-date=2021-06-21|website=Fusion Industry Assn|language=en|archive-date=2023-04-20 |archive-url=https://web.archive.org/web/20230420002958/https://www.fusionindustryassociation.org/post/uk-s-regulatory-horizons-council-issues-report-on-fusion-energy-regulation|url-status=dead}}</ref> This call was met by the UK government publishing in October 2021 both its ''Fusion Green Paper'' and its ''Fusion Strategy'', to regulate and commercialize fusion, respectively.<ref>{{Cite book |url=https://assets.publishing.service.gov.uk/government/uploads/system/uploads/attachment_data/file/1022540/towards-fusion-energy-uk-government-fusion-strategy.pdf |title=Towards Fusion Energy: The UK Government's Fusion Strategy |publisher=UK Government, Department for Business, Energy, & Industrial Strategy |year=2021 |location=London, UK |language=En}}</ref><ref>{{Cite web|title=Government sets out vision for UK's rollout of commercial fusion energy|url=https://www.gov.uk/government/news/government-sets-out-vision-for-uks-rollout-of-commercial-fusion-energy|access-date=2021-10-15|website=GOV.UK|language=en}}</ref><ref>{{Cite web|title=UK government publishes fusion strategy – Nuclear Engineering International|url=https://www.neimagazine.com/news/newsuk-government-publishes-fusion-strategy-9129061|access-date=2021-10-15|website=www.neimagazine.com|date=October 5, 2021 }}</ref> Then, in April 2023, in a decision likely to influence other nuclear regulators, the NRC announced in a unanimous vote that fusion energy would be regulated not as fission but under the same regulatory regime as particle accelerators.<ref>{{Cite web |last=Holland |first=Andrew |date=2023-04-14 |title=NRC Decision Separates Fusion Energy Regulation from Nuclear Fission |url=https://www.fusionindustryassociation.org/post/nrc-decision-separates-fusion-energy-regulation-from-nuclear-fission |access-date=2023-04-19 |website=Fusion Industry Assn |language=en}}</ref> |
|||
[[Image:Fusion target implosion on NOVA laser.jpg|thumb|right|200px|Inertial confinement fusion implosion on the [[Nova laser]] creates "microsun" conditions of tremendously high density and temperature.]] |
|||
Although the standard solution for electricity production in fusion power plant designs is conventional steam turbines using the heat deposited by neutrons, there are also designs for direct conversion of the energy of the charged particles into electricity. These are of little value with a D-T fuel cycle, where 80% of the power is in the neutrons, but are indispensable with [[aneutronic fusion]], where less than 1% is. Direct conversion has been most commonly proposed for open-ended magnetic configurations like [[magnetic mirror]]s or [[Field-Reversed Configuration]]s, where charged particles are lost along the magnetic field lines, which are then expanded to convert a large fraction of the random energy of the fusion products into directed motion. The particles are then collected on electrodes at various large electrical potentials. Typically the claimed conversion efficiency is in the range of 80%, but the converter may approach the reactor itself in size and expense. |
|||
Then, in October 2023 the UK government, in enacting the Energy Act 2023, made the UK the first country to legislate for fusion separately from fission, to support planning and investment, including the UK's planned prototype fusion power plant for 2040; [[Spherical Tokamak for Energy Production|STEP]]<ref>{{Cite web |title=New laws passed to bolster energy security and deliver net zero |url=https://www.gov.uk/government/news/new-laws-passed-to-bolster-energy-security-and-deliver-net-zero |access-date=2023-11-10 |website=GOV.UK |language=en}}</ref> the UK is working with Canada and Japan in this regard.<ref>{{Cite web |title=Agile Nations: UK, Japan and Canada joint recommendations on fusion energy |url=https://www.gov.uk/government/publications/agile-nations-uk-japan-and-canada-joint-recommendations-on-fusion-energy |access-date=2024-03-20 |website=GOV.UK |language=en}}</ref> Meanwhile, in February 2024 the US House of Representatives passed the Atomic Energy Advancement Act, which includes the Fusion Energy Act, which establishes a regulatory framework for fusion energy systems.<ref>{{Cite web |date=2024-02-29 |title=Fusion Caucus Celebrates House Passage of Bipartisan Fusion Energy Act |url=https://beyer.house.gov/news/documentsingle.aspx?DocumentID=6086 |access-date=2024-03-01 |website=U.S. Representative Don Beyer |language=en}}</ref> |
|||
==Safety and the environment== |
|||
===Accident potential=== |
|||
{{Unreferenced section|date=March 2011}} |
|||
There is no possibility of a ''catastrophic'' accident in a fusion reactor resulting in major release of radioactivity to the environment or injury to non-staff, unlike modern fission reactors. The primary reason is that nuclear fusion requires precisely controlled temperature, pressure, and magnetic field parameters to generate net energy. If the reactor were damaged, these parameters would be disrupted and the heat generation in the reactor would rapidly cease. In contrast, the fission products in a fission reactor continue to generate heat through [[beta-decay]] for several hours or even days after reactor shut-down, meaning that melting of fuel rods is possible even after the reactor has been stopped due to continued accumulation of heat. |
|||
== Geopolitics == |
|||
There is also no risk of a runaway reaction in a fusion reactor, since the [[plasma (physics)|plasma]] is normally burnt at optimal conditions, and any significant change will render it unable to produce excess heat. In fusion reactors the reaction process is so delicate that this level of safety is inherent; no elaborate failsafe mechanism is required. Although the plasma in a fusion power plant will have a volume of 1000 cubic meters or more, the density of the plasma is extremely low, and the total amount of fusion fuel in the vessel is very small, typically a few grams. If the fuel supply is closed, the reaction stops within seconds. In comparison, a fission reactor is typically loaded with enough fuel for one or several years, and no additional fuel is necessary to keep the reaction going. |
|||
Given the potential of fusion to transform the world's [[energy industry]] and mitigate [[climate change]],<ref name="regulation">{{Cite web|last=Holland|first=Andrew|title=Fusion energy needs smart federal government regulation|url=https://www.washingtontimes.com/news/2020/oct/6/fusion-energy-needs-smart-federal-government-regul/|access-date=2020-10-10|website=The Washington Times|language=en-US}}</ref><ref>{{Cite web|last=Turrell|first=Arthur|date=2021-08-28|title=The race to give nuclear fusion a role in the climate emergency|url=http://www.theguardian.com/environment/2021/aug/28/the-race-to-give-nuclear-fusion-a-role-in-the-climate-emergency|access-date=2022-02-15|website=the Guardian|language=en}}</ref> fusion science has traditionally been seen as an integral part of peace-building [[science diplomacy]].<ref name="quest">{{Cite book|author1=Clery, Daniel|title=A piece of the sun: the quest for fusion energy |date=2014|isbn=978-1468310412|location=New York |publisher=Overlook Duckworth |oclc=1128270426}}</ref><ref name="ITER">{{Cite book|last=Claessens, Michel |title=ITER: the giant fusion reactor: bringing a sun to Earth|date= 2019|isbn=978-3030275815|location=Cham |publisher=Springer |oclc=1124925935}}</ref> However, technological developments<ref>{{Cite news|date=2018-04-18|title=Will China beat the world to nuclear fusion and clean energy?|language=en-GB |publisher=BBC News |work=China Blog |url=https://www.bbc.com/news/blogs-china-blog-43792655|access-date=2020-10-12}}</ref> and private sector involvement has raised concerns over intellectual property, regulatory administration, global leadership;<ref name="regulation" /> equity, and potential weaponization.<ref name="Diffusion">{{Cite journal|last1=Carayannis|first1=Elias G.|last2=Draper|first2=John|last3=Iftimie|first3=Ion A.|date=2020|title=Nuclear Fusion Diffusion: Theory, Policy, Practice, and Politics Perspectives|url=https://ieeexplore.ieee.org/document/9078039|journal=IEEE Transactions on Engineering Management|volume=69 |issue=4 |pages=1237–1251|doi=10.1109/TEM.2020.2982101|s2cid=219001461|issn=0018-9391}}</ref><ref name="Urgent Action">{{Cite journal|last1=Carayannis|first1=Elias G.|last2=Draper|first2=John|last3=Bhaneja|first3=Balwant|date=2020-10-02|title=Towards Fusion Energy in the Industry 5.0 and Society 5.0 Context: Call for a Global Commission for Urgent Action on Fusion Energy|journal=Journal of the Knowledge Economy|volume=12|issue=4|pages=1891–1904|language=en|doi=10.1007/s13132-020-00695-5|issn=1868-7873|s2cid=222109349|doi-access=free}}</ref> These challenge ITER's peace-building role and led to calls for a global commission.<ref name="Urgent Action" /><ref>{{Cite journal|last1=Carayannis|first1=Elias G.|last2=Draper|first2=John|date=2021-04-22|title=The place of peace in the ITER machine assembly launch: Thematic analysis of the political speeches in the world's largest science diplomacy experiment.|url=http://dx.doi.org/10.1037/pac0000559|journal=Peace and Conflict: Journal of Peace Psychology|volume=27|issue=4|pages=665–668|doi=10.1037/pac0000559|s2cid=235552703|issn=1532-7949}}</ref> Fusion power significantly contributing to climate change by 2050 seems unlikely without substantial breakthroughs and a space race mentality emerging,<ref name="Tokamak" /><ref>{{Cite journal|last1=Gi|first1=Keii|last2=Sano|first2=Fuminori|last3=Akimoto|first3=Keigo|last4=Hiwatari|first4=Ryoji|last5=Tobita|first5=Kenji|date=2020|title=Potential contribution of fusion power generation to low-carbon development under the Paris Agreement and associated uncertainties|journal=Energy Strategy Reviews |language=en|volume=27|pages=100432|doi=10.1016/j.esr.2019.100432|doi-access=free|bibcode=2020EneSR..2700432G }}</ref> but a contribution by 2100 appears possible, with the extent depending on the type and particularly cost of technology pathways.<ref>{{Cite journal |last1=Nicholas |first1=T. E. G. |last2=Davis |first2=T. P. |last3=Federici |first3=F. |last4=Leland |first4=J. |last5=Patel |first5=B. S. |last6=Vincent |first6=C. |last7=Ward |first7=S. H. |date=2021 |title=Re-examining the role of nuclear fusion in a renewables-based energy mix |url=http://dx.doi.org/10.1016/j.enpol.2020.112043 |journal=Energy Policy |volume=149 |pages=112043 |arxiv=2101.05727 |doi=10.1016/j.enpol.2020.112043 |bibcode=2021EnPol.14912043N |issn=0301-4215 |s2cid=230570595}}</ref><ref>{{Cite journal|last1=Carayannis|first1=Elias|last2=Draper|first2=John|last3=Crumpton|first3=Charles|date=2022|title=Reviewing fusion energy to address climate change by 2050|url=https://www.scribd.com/document/557471226/Reviewing-Fusion-Energy-to-Address-Climate-Change-by-2050-by-Elias-G-Carayannis-John-Draper-and-Charles-David-Crumpton|journal=Journal of Energy and Development|volume=47|issue=1}}</ref> |
|||
Developments from late 2020 onwards have led to talk of a "new space race" with multiple entrants, pitting the US against China<ref name=5big>{{Cite journal|last=Clynes|first=Tom|date=2020|title=5 Big ideas for fusion power: Startups, universities, and major companies are vying to commercialize a nuclear fusion reactor|url=https://ieeexplore.ieee.org/document/8976899|journal=IEEE Spectrum|volume=57|issue=2|pages=30–37|doi=10.1109/MSPEC.2020.8976899|s2cid=211059641|issn=0018-9235}}</ref> and the UK's [[Spherical Tokamak for Energy Production|STEP]] FPP.<ref>{{Cite web|date=2021-04-14|title=National Academies calls for a fusion pilot plant|url=https://thebulletin.org/2021/04/national-academies-calls-for-a-fusion-pilot-plant/|access-date=2021-04-15|website=Bulletin of the Atomic Scientists|language=en-US}}</ref><ref>{{Cite web|date=2021-07-13|title=US must make an infrastructure investment in fusion energy|url=https://www.washingtonexaminer.com/opinion/op-eds/us-must-make-an-infrastructure-investment-in-fusion-energy|access-date=2021-07-16|website=Washington Examiner|language=en}}</ref> On 24 September 2020, the United States House of Representatives approved a research and commercialization program. The Fusion Energy Research section incorporated a milestone-based, cost-sharing, [[public-private partnership]] program modeled on [[NASA]]'s COTS program, which launched the commercial [[space industry]].<ref name="Space Race" /> In February 2021, the National Academies published ''Bringing Fusion to the U.S. Grid'', recommending a market-driven, cost-sharing plant for 2035–2040,<ref>{{Cite web|title=An aggressive market-driven model for US fusion power development|url=https://news.mit.edu/2021/aggressive-market-driven-model-us-fusion-power-development-0224|access-date=2021-02-26|website=MIT News {{!}} Massachusetts Institute of Technology|date=February 24, 2021 |language=en}}</ref><ref>{{Cite web|last1=Cho |first1=Adrian |date=2021-02-19|title=Road map to U.S. fusion power plant comes into clearer focus – sort of|url=https://www.science.org/content/article/road-map-us-fusion-power-plant-comes-clearer-focus-sort|access-date=2021-03-06|website=Science |language=en}}</ref><ref>{{Cite journal |last=Kramer |first=David |date=2021-03-10|title=Academies urge public–private effort to build a pilot fusion-power plant |journal=Physics Today |volume= 2021|issue=2 |pages= 0310a|language=en|doi=10.1063/PT.6.2.20210310a|s2cid=243296520 |doi-access=free|bibcode=2021PhT..2021b.310. }}</ref> and the launch of the Congressional Bipartisan Fusion Caucus followed.<ref>{{Cite web |date=2021-02-19 |title=FIA Congratulates Congressional Bipartisan Fusion Caucus |url=https://www.fusionindustryassociation.org/post/fia-congratulates-congressional-bipartisan-fusion-caucus |access-date=2021-02-26 |website=Fusion Industry Association |language=en-us}}</ref> |
|||
In the magnetic approach, strong fields are developed in coils that are held in place mechanically by the reactor structure. Failure of this structure could release this tension and allow the magnet to "explode" outward. The severity of this event would be similar to any other industrial accident or an [[MRI]] machine [http://www.indyrad.iupui.edu/RadWeb/Portals/0/ContentFiles/Education/Training/MR_Safety_files/frame.htm quench]/explosion, and could be effectively stopped with a [[containment building]] similar to those used in existing (fission) nuclear generators. The laser-driven inertial approach is generally lower-stress. Although failure of the reaction chamber is possible, simply stopping fuel delivery would prevent any sort of catastrophic failure. |
|||
In December 2020, an independent expert panel reviewed [[EUROfusion]]'s design and R&D work on DEMO, and EUROfusion confirmed it was proceeding with its Roadmap to Fusion Energy, beginning the conceptual design of DEMO in partnership with the European fusion community, suggesting an EU-backed machine had entered the race.<ref>{{Cite web|last=Vries|first=Gieljan de|title=Expert panel approves next DEMO design phase|url=https://www.euro-fusion.org/news/2020/december/expert-panel-approves-next-demo-design-phase/|access-date=2021-02-16|website=www.euro-fusion.org|date=December 15, 2020 |language=en}}</ref> |
|||
Most reactor designs rely on the use of liquid [[lithium]] as both a coolant and a method for converting stray neutrons from the reaction into [[tritium]], which is fed back into the reactor as fuel. Lithium is highly flammable, and in the case of a fire it is possible that the lithium stored on-site could be burned up and escape. In this case the tritium contents of the lithium would be released into the atmosphere, posing a radiation risk. However, calculations suggest that the total amount of tritium and other radioactive gases in a typical power plant would be so small, about 1 kg, that they would have diluted to legally acceptable limits by the time they blew as far as the plant's [[perimeter fence]].<ref name="WorldEnergyCouncil"/> |
|||
In November 2023 the UK and the US announced a bilateral partnership to accelerate fusion energy. Then, in December 2023 at [[2023 United Nations Climate Change Conference|COP28]] the US announced a US global strategy to commercialize fusion energy.<ref>{{Cite web |date=2023-12-05 |title=At COP28, John Kerry unveils nuclear fusion strategy as a source of clean energy |url=https://apnews.com/article/fusion-nuclear-john-kerry-cop28-climate-power-energy-40ffa257eae528163f68554368cacfee |access-date=2023-12-08 |website=AP News |language=en}}</ref> Then, in April 2024, Japan and the US announced a similar partnership,<ref>{{Cite news |last1=Renshaw |first1=Jarrett |last2=Gardner |first2=Timothy |date=10 April 2024 |title=US, Japan announce partnership to accelerate nuclear fusion |url=https://www.reuters.com/business/energy/us-japan-announce-joint-partnership-accelerate-nuclear-fusion-sources-2024-04-10/ |work=Reuters}}</ref> and in May of the same year, the G7 announced a G7 Working Group on Fusion Energy to promote international collaborations to accelerate the development of commercial energy and promote R&D between countries, as well as rationalize fusion regulation.<ref>{{Cite web |last=Caroline |date=2024-04-30 |title=G7 Puts Fusion Forward At The Climate, Energy And Environment Ministers' Meeting |url=https://www.fusionindustryassociation.org/g7-puts-fusion-forward-at-the-climate-energy-and-environment-ministers-meeting/ |access-date=2024-05-11 |website=Fusion Industry Association |language=en-US}}</ref> |
|||
The likelihood of ''small industrial'' accidents including the local release of radioactivity and injury to staff cannot be estimated yet. These would include accidental releases of lithium, tritium, or mis-handling of decommissioned radioactive components of the reactor itself. |
|||
Specifically to resolve the tritium supply problem, in February 2024, the UK ([[United Kingdom Atomic Energy Authority|UKAEA]]) and Canada ([[Canadian Nuclear Laboratories Research Facilities|Canadian Nuclear Laboratories]]) announced an agreement by which Canada could refurbish its [[CANDU reactor|Candu]] deuterium-uranium tritium-generating heavywater nuclear plants and even build new ones, guaranteeing a supply of tritium into the 2070s, while the UKAEA would test breeder materials and simulate how tritium could be captured, purified, and injected back into the fusion reaction.<ref>{{Cite web |title=UK and Canada team up to solve nuclear fusion fuel shortage |url=https://sciencebusiness.net/news/uk-and-canada-team-solve-nuclear-fusion-fuel-shortage |access-date=2024-05-11 |website=Science{{!}}Business |language=en}}</ref> |
|||
===Effluents during normal operation=== |
|||
The natural product of the fusion reaction is a small amount of [[helium]], which is completely harmless to life. Of more concern is [[tritium]], which, like other isotopes of hydrogen, is difficult to retain completely. During normal operation, some amount of tritium will be continually released. There would be no acute danger, but the cumulative effect on the world's population from a fusion economy could be a matter of concern.{{Citation needed|date=June 2007}} |
|||
== Advantages == |
|||
Although tritium is volatile and biologically active, the health risk posed by a release is much lower than that of most radioactive contaminants, due to tritium's short half-life (12 years), very low decay energy (~14.95 keV), and the fact that it does not [[bioaccumulation|bioaccumulate]] (instead being cycled out of the body as water, with a [[biological half-life]] of 7 to 14 days).<ref name="nuclearsafety-petrangeli">{{cite book |title=Nuclear Safety |last=Petrangeli |first=Gianni |year=2006 |publisher=Butterworth-Heinemann |isbn=9780750667234 |page=226 |pages=430 |accessdate=}}</ref> Current ITER designs are investigating total containment facilities for any tritium. |
|||
Fusion power promises to provide more energy for a given weight of fuel than any fuel-consuming energy source currently in use.<ref>{{cite web |author=Heeter |first=Robert F. |display-authors=etal |title=Conventional Fusion FAQ Section 2/11 (Energy) Part 2/5 (Environmental) |url=http://fusedweb.llnl.gov/FAQ/section2-energy/part2-enviro.txt |url-status=dead |archive-url=https://web.archive.org/web/20010303051913/http://fusedweb.llnl.gov/FAQ/section2-energy/part2-enviro.txt |archive-date=3 March 2001 |access-date=30 October 2014 |publisher=Fused.web.llnl.gov}}</ref> The fuel (primarily [[deuterium]]) exists abundantly in the ocean: about 1 in 6500 hydrogen atoms in seawater is deuterium.<ref>{{cite web |author=Stadermann |first=Frank J. |title=Relative Abundances of Stable Isotopes |url=http://presolar.wustl.edu/work/abundances.html |archive-url=https://web.archive.org/web/20110720122226/http://presolar.wustl.edu/work/abundances.html |archive-date=2011-07-20 |publisher=Laboratory for Space Sciences, Washington University in St. Louis}}</ref> Although this is only about 0.015%, seawater is plentiful and easy to access, implying that fusion could supply the world's energy needs for millions of years.<ref>{{cite web |last1=Ongena |first1=J. |last2=Van Oost |first2=G. |title=Energy for Future Centuries |url=http://www.agci.org/dB/PDFs/03S2_MMauel_SafeFusion%3F.pdf |url-status=dead |archive-url=https://web.archive.org/web/20110727135814/http://www.agci.org/dB/PDFs/03S2_MMauel_SafeFusion?.pdf |archive-date=2011-07-27 |publisher=Laboratorium voor Plasmafysica – Laboratoire de Physique des Plasmas Koninklijke Militaire School – École Royale Militaire; Laboratorium voor Natuurkunde, Universiteit Gent |pages=Section III.B. and Table VI}}</ref><ref>{{cite web|url=http://www.eps.org/about-us/position-papers/fusion-energy/|archive-url=https://web.archive.org/web/20081008001417/http://www.eps.org/about-us/position-papers/fusion-energy/|archive-date=2008-10-08|title=The importance of European fusion energy research|publisher=The European Physical Society|author=EPS Executive Committee}}</ref> |
|||
First generation fusion plants are expected to use the deuterium-tritium fuel cycle. This will require the use of lithium for breeding of the tritium. It is not known for how long global lithium supplies will suffice to supply this need as well as those of the battery and metallurgical industries. It is expected that second generation plants will move on to the more formidable deuterium-deuterium reaction. The deuterium-helium-3 reaction is also of interest, but the light helium isotope is practically non-existent on Earth. It is thought to exist in useful quantities in the [[lunar regolith]], and is abundant in the atmospheres of the gas giant planets. |
|||
===Waste management=== |
|||
The large flux of high-energy neutrons in a reactor will make the structural materials radioactive. The radioactive inventory at shut-down may be comparable to that of a fission reactor, but there are important differences. |
|||
Fusion power could be used for so-called "deep space" propulsion within the solar system<ref>{{Cite web|title=Space propulsion {{!}} Have fusion, will travel|url=http://www.iter.org/newsline/-/3303|access-date=2021-06-21|website=ITER|language=en}}</ref><ref>{{Cite web|last=Holland|first=Andrew|date=2021-06-15|title=Funding for Fusion for Space Propulsion|url=https://www.fusionindustryassociation.org/post/fia-proposes-funding-for-fusion-for-space-propulsion|access-date=2021-06-21|website=Fusion Industry Assn|language=en|archive-date=2023-04-20 |archive-url=https://web.archive.org/web/20230420002956/https://www.fusionindustryassociation.org/post/fia-proposes-funding-for-fusion-for-space-propulsion|url-status=dead}}</ref> and for [[interstellar space]] exploration where solar energy is not available, including via [[Antimatter-catalyzed nuclear pulse propulsion|antimatter-fusion]] hybrid drives.<ref>{{Cite book|last1=Schulze|first1=Norman R. |title=Fusion energy for space missions in the 21st century|last2=United States|last3=National Aeronautics and Space Administration|last4=Scientific and Technical Information Program|date=1991|publisher=National Aeronautics and Space Administration, Office of Management, Scientific and Technical Information Program; [For sale by the National Technical Information Service [distributor|location=Washington, DC; Springfield, Va.|language=en|oclc=27134218}}</ref><ref>{{Cite book |chapter=Principles of Fusion Energy Utilization in Space Propulsion|date=1995-01-01|url=https://arc.aiaa.org/doi/10.2514/5.9781600866357.0001.0046 |title=Fusion Energy in Space Propulsion|pages=1–46|series=Progress in Astronautics and Aeronautics|publisher=American Institute of Aeronautics and Astronautics|doi=10.2514/5.9781600866357.0001.0046|isbn=978-1563471841|access-date=2020-10-11 }}</ref> |
|||
The half-life of the [[radioisotopes]] produced by fusion tend to be less than those from fission, so that the inventory decreases more rapidly. Unlike fission reactors, whose waste remains radioactive for thousands of years, most of the radioactive material in a fusion reactor would be the reactor core itself, which would be dangerous for about 50 years, and low-level waste another 100. Although this waste will be considerably more radioactive during those 50 years than fission waste, the very short half-life makes the process very attractive, as the waste management is fairly straightforward. By 300 years the material would have the same radioactivity as [[coal ash]].<ref name="WorldEnergyCouncil">{{Cite web |
|||
|url=http://www.worldenergy.org/wec-geis/publications/default/tech_papers/18th_Congress/downloads/ds/ds6/ds6_5.pdf |
|||
|format=PDF|title=Fusion as a Future Power Source: Recent Achievements and Prospects |
|||
|author=T. Hamacher and A.M. Bradshaw |
|||
|publisher=World Energy Council |
|||
|year=2001 |
|||
|month=October |
|||
|archiveurl=http://web.archive.org/web/20040506065141/http://www.worldenergy.org/wec-geis/publications/default/tech_papers/18th_Congress/downloads/ds/ds6/ds6_5.pdf |
|||
|archivedate=2004-05-06 |
|||
}}</ref> |
|||
== Disadvantages == |
|||
Additionally, the choice of materials used in a fusion reactor is less constrained than in a fission design, where many materials are required for their specific [[neutron cross-section]]s. This allows a fusion reactor to be designed using materials that are selected specifically to be "low activation", materials that do not easily become radioactive. [[Vanadium]], for example, would become much less radioactive than [[stainless steel]]. [[Carbon fiber]] materials are also low-activation, as well as being strong and light, and are a promising area of study for laser-inertial reactors where a magnetic field is not required. |
|||
Fusion power has a number of disadvantages. Because 80 percent of the energy in any reactor fueled by deuterium and tritium appears in the form of neutron streams, such reactors share many of the drawbacks of fission reactors. This includes the production of large quantities of [[#Radioactive waste|radioactive waste]] and serious radiation damage to reactor components. Additionally, naturally occurring tritium is extremely rare. While the hope is that fusion reactors can breed their own tritium, tritium self-sufficiency is extremely challenging, not least because tritium is difficult to contain (tritium has leaked from 48 of 65 nuclear sites in the US<ref> |
|||
{{Cite web|url=https://www.nbcnews.com/id/wbna43475479|title=Radioactive tritium leaks found at 48 US nuke sites|last=Donn|first=Jeff|date=2011-06-21|access-date=2023-07-04|archive-url=https://web.archive.org/web/20201111214625/https://www.nbcnews.com/id/wbna43475479|archive-date=2020-11-11|publisher=[[NBC News]] |language=en-US}}</ref>). In any case the reserve and start-up tritium inventory requirements are likely to be unacceptably large.<ref name="TritiumSelfSufficiency_Abdou">{{cite journal |last1=Abdou |first1=M. |display-authors=et al |year=2020 |title=Physics and technology considerations for the deuterium-tritium fuel cycle and conditions for tritium fuel self sufficiency |url=https://iopscience.iop.org/article/10.1088/1741-4326/abbf35 |journal=Nuclear Fusion |volume=61 |issue=1 |page=013001 |doi=10.1088/1741-4326/abbf35|s2cid=229444533 }}</ref> |
|||
If reactors can be made to operate using only deuterium fuel, then the tritium replenishment issue is eliminated and neutron radiation damage may be reduced. However, the probabilities of deuterium-deuterium reactions are about 20 times lower than for deuterium-tritium. Additionally, the temperature needed is about 3 times higher than for deuterium-tritium (see [[#Cross section|cross section]]). The higher temperatures and lower reaction rates thus significantly complicate the engineering challenges. In any case, other drawbacks remain, for instance reactors requiring only deuterium fueling will have greatly enhanced [[#Nuclear proliferation|nuclear weapons proliferation potential]]. |
|||
In general terms, fusion reactors would create far less radioactive material than a fission reactor, the material it would create is less damaging biologically, and the radioactivity "burns off" within a time period that is well within existing engineering capabilities.<ref>Basu, S. K. ''Encyclopaedic Dictionary of Astrophysics''. Global Vision, 2007, pg. 110</ref> |
|||
== History == |
|||
===Nuclear proliferation=== |
|||
{{Main|History of nuclear fusion}} |
|||
Although fusion power uses nuclear technology, the overlap with nuclear weapons technology is small. [[Tritium]] is a component of the trigger of [[hydrogen bomb]]s, but not a major problem in production. The copious neutrons from a fusion reactor could be used to breed [[plutonium]] for an atomic bomb, but not without extensive redesign of the reactor, so that production would be difficult to conceal. The theoretical and computational tools needed for hydrogen bomb design are closely related to those needed for [[inertial confinement fusion]], but have very little in common with the more scientifically developed [[magnetic confinement fusion]]. |
|||
{{cleanup rewrite|2=section|date=February 2023}} |
|||
=== |
=== Early experiments === |
||
[[File:Kink instability at Aldermaston.jpg|thumb|right|upright=1.5|Early photo of plasma inside a pinch machine (Imperial College 1950–1951)]] |
|||
Large-scale reactors using neutronic fuels (e.g. [[ITER]]) and thermal power production (turbine based) are most comparable to [[nuclear power|fission power]] from an engineering and economics viewpoint. Both fission and fusion power plants involve a relatively compact heat source powering a conventional steam turbine-based power plant, while producing enough neutron radiation to make [[neutron activation|activation]] of the plant materials problematic. The main distinction is that fusion power produces no high-level radioactive waste (though activated plant materials still need to be disposed of). There are some power plant ideas which may significantly lower the cost or size of such plants; however, research in these areas is nowhere near as advanced as in [[tokamak]]s. |
|||
[[File:A sun of our own newspaper headline.jpg|thumb|alt=The UK claimed that it had gotten fusion first in 1957 on ZETA, but this claim had to later be withdrawn. |The UK claimed that it had gotten fusion first in 1957 on ZETA, but this claim had to later be withdrawn.]] |
|||
The first machine to achieve controlled [[thermonuclear fusion]] was a [[Pinch (plasma physics)|pinch machine]] at Los Alamos National Laboratory called Scylla I at the start of 1958. The team that achieved it was led by a British scientist named [[James L. Tuck|James Tuck]] and included a young [[Marshall Rosenbluth]]. Tuck had been involved in the Manhattan project, but had switched to working on fusion in the early 1950s. He applied for funding for the project as part of a White House sponsored contest to develop a fusion reactor along with [[Lyman Spitzer]]. Spitzer went on to develop the Stellarator concept at Princeton. The previous year, 1957, the British had claimed that they had achieved thermonuclear fusion reactions on the [[ZETA (fusion reactor)|Zeta pinch machine]]. However, it turned out that the neutrons they had detected were from beam-target interactions, not fusion, and they withdrew the claim. |
|||
Fusion power commonly proposes the use of [[deuterium]], an [[isotope]] of hydrogen, as fuel and in many current designs also use [[lithium]]. Assuming a fusion energy output equal to the 1995 global power output of about 100 [[exa-|E]]J/yr (= 1 x 10<sup>20</sup> J/yr) and that this does not increase in the future, then the known current lithium reserves would last 3000 years, lithium from sea water would last 60 million years, and a more complicated fusion process using only deuterium from sea water would have fuel for 150 billion years.<ref>[http://www.agci.org/dB/PDFs/03S2_MMauel_SafeFusion%3F.pdf Energy for Future Centuries]</ref> To put this in context, 150 billion years is over ten times the currently measured age of the universe, and is close to 30 times the remaining lifespan of the sun.<ref name="sunlife">{{Cite web|url=http://helios.gsfc.nasa.gov/qa_sun.html#sunlife|title=Cosmicopia|last=Dr. Eric Christian, Et al.|publisher=NASA|accessdate=2009-03-20}}</ref> |
|||
Scylla I was a classified machine at the time, so the fact that humankind had demonstrated controlled nuclear fusion in bulk was hidden from the public. A traditional Z-pinches passes a current down the center of a plasma, which makes a magnetic force around the outside which squeezes the plasma to fusion conditions. Scylla I was a twist on this basic idea; it was a θ-pinch, which passes a current around the outside creating a magnetic force in the center <ref name="Phillips, James 2013" /> Scylla I was a θ-pinch machine, with a cylinder full of deuterium.<ref name="Seife, Charles 2008" /><ref name="Phillips, James 2013" /> After the success of Scylla-I, Los Alamos went on to build multiple pinch machines over the next few years. But the problem with pinches were that they were unstable because of a whole host of instabilities. |
|||
==Economics== |
|||
While fusion power is still in early stages of development, substantial sums have been and continue to be invested in research. In the EU almost € 10 billion was spent on fusion research up to the end of the 1990s, and the new [[ITER]] reactor alone is budgeted at € 10 billion. It is estimated that up to the point of possible implementation of electricity generation by nuclear fusion, R&D will need further promotion totalling around € 60-80 billion over a period of 50 years or so (of which € 20-30 billion within the EU).<ref>{{Cite web| title=The current EU research programme | url=http://www.tab.fzk.de/en/projekt/zusammenfassung/ab75.htm | work=[[Sixth Framework Programme|FP6]] | publisher= | date= | accessdate= }}</ref> Nuclear fusion research receives € 750 million (excluding ITER funding), compared with € 810 million for all non-nuclear energy research combined,<ref>{{Cite web| title=The Sixth Framework Programme in brief | url=http://ec.europa.eu/research/fp6/pdf/fp6-in-brief_en.pdf | work= | publisher= | date= | accessdate= }}</ref> putting research into fusion power well ahead of that of any single rivaling technology. |
|||
Lots of fusion approaches were pursued in parallel during this period and Princeton emerged as a hub for Stellarator research. It was pioneered by [[Lyman Spitzer]]. While fusion did not immediately transpire, the effort led to the creation of the [[Princeton Plasma Physics Laboratory]].<ref>{{Cite journal|last=Stix|first=T. H.|date=1998|title=Highlights in early stellarator research at Princeton|url=http://inis.iaea.org/Search/search.aspx?orig_q=RN:30002355|journal=Helical System Research|language=en}}</ref><ref>{{Cite tech report|last=Johnson|first=John L.|date=2001-11-16|title=The Evolution of Stellarator Theory at Princeton|url=https://www.osti.gov/biblio/792587-fxKdXU/native/|language=en|doi=10.2172/792587|osti=792587}}</ref> |
|||
==Advantages== |
|||
Fusion power would provide much more energy for a given weight of fuel than any technology currently in use,<ref>{{Cite web |
|||
|url=http://fusedweb.llnl.gov/FAQ/section2-energy/part2-enviro.txt |
|||
|title=Conventional Fusion FAQ Section 2/11 (Energy) Part 2/5 (Environmental) |
|||
|author=Robert F. Heeter, et al.}}</ref> and the fuel itself (primarily [[deuterium]]) exists abundantly in the Earth's ocean: about 1 in 6500 hydrogen atoms in seawater is deuterium.<ref>{{Cite web |
|||
|url=http://presolar.wustl.edu/work/abundances.html |
|||
|title=Relative Abundances of Stable Isotopes |
|||
|author=Dr. Frank J. Stadermann |
|||
|publisher=Laboratory for Space Sciences, Washington University in St. Louis |
|||
}}</ref> Although this may seem a low proportion (about 0.015%), because nuclear fusion reactions are so much more energetic than chemical combustion and seawater is easier to access and more plentiful than fossil fuels, fusion could potentially supply the world's energy needs for millions of years.<ref>{{Cite web |
|||
|url=http://www.agci.org/dB/PDFs/03S2_MMauel_SafeFusion%3F.pdf |
|||
|format=PDF|title=Energy for Future Centuries |
|||
|author=J. Ongena and G. Van Oost |
|||
|publisher=Laboratorium voor Plasmafysica– Laboratoire de Physique des Plasmas |
|||
Koninklijke Militaire School– Ecole Royale Militaire; Laboratorium voor Natuurkunde, Universiteit Gent |
|||
|pages=Section III.B. and Table VI |
|||
}}</ref><ref>{{Cite web |
|||
|url=http://www.eps.org/about-us/position-papers/fusion-energy/ |
|||
|title=The importance of European fusion energy research |
|||
|publisher=The European Physical Society |
|||
|author=EPS Executive Committee |
|||
}}</ref> |
|||
=== First tokamak === |
|||
Despite being technically [[non-renewable energy|non-renewable]], fusion power has many of the benefits of renewable energy sources (such as being a long-term energy supply and emitting no [[greenhouse gas]]es) as well as some of the benefits of the resource-limited energy sources as hydrocarbons and nuclear fission (without [[Nuclear reprocessing|reprocessing]]). Like these currently dominant energy sources, fusion could provide very high power-generation density and uninterrupted power delivery (due to the fact that it is not dependent on the [[weather]], unlike wind and solar power). |
|||
The concept of the tokamak originated in 1950–1951 from [[Igor Tamm|I.E. Tamm]] and [[Andrei Sakharov|A.D. Sakharov]] in the [[Soviet Union]]. The tokamak essentially combined a low-power pinch device with a low-power stellarator.<ref name="quest" /> |
|||
[[Andrei Sakharov|A.D. Sakharov]]'s group constructed the first tokamaks, achieving the first quasistationary fusion reaction.<ref>{{Cite book|last=Irvine|first=Maxwell |title=Nuclear power: a very short introduction|date=2014|publisher=Oxford University Press|isbn=978-0199584970|location=Oxford|language=en|oclc=920881367}}</ref><sup>:90</sup> |
|||
Over time the "advanced tokamak" concept emerged, which included non-circular plasma, internal diverters and limiters, superconducting magnets, operation in the so-called "H-mode" island of increased stability,<ref>{{Citation|last=Kusama|first=Y.|title=Requirements for Diagnostics in Controlling Advanced Tokamak Modes|date=2002|work=Advanced Diagnostics for Magnetic and Inertial Fusion|pages=31–38|editor-last=Stott|editor-first=Peter E.|place=Boston, MA|publisher=Springer US|language=en|doi=10.1007/978-1-4419-8696-2_5|isbn=978-1441986962|editor2-last=Wootton|editor2-first=Alan|editor3-last=Gorini|editor3-first=Giuseppe|editor4-last=Sindoni|editor4-first=Elio}}</ref> and the compact tokamak, with the magnets on the inside of the vacuum chamber.<ref>{{Cite journal|last=Menard|first=J. E.|date=2019-02-04|title=Compact steady-state tokamak performance dependence on magnet and core physics limits|url= |journal=Philosophical Transactions of the Royal Society A: Mathematical, Physical and Engineering Sciences|volume=377|issue=2141|pages=20170440|doi=10.1098/rsta.2017.0440|pmid=30967044|pmc=6365855|bibcode=2019RSPTA.37770440M|issn=1364-503X}}</ref><ref>{{Cite journal |last=Kaw |first=P. K. |date=1999 |title=Steady state operation of tokamaks |journal=Nuclear Fusion |volume=39 |issue=11 |pages=1605–1607 |doi=10.1088/0029-5515/39/11/411 |issn=0029-5515 |s2cid=250826481}}</ref> |
|||
Another aspect of fusion energy is that the cost of production does not suffer from [[diseconomies of scale]]. The cost of water and wind energy, for example, goes up as the optimal locations are developed first, while further generators must be sited in less ideal conditions. With fusion energy, the production cost will not increase much, even if large numbers of plants are built{{Citation needed|date=April 2011}}. |
|||
[[File:TMX Baseball Coils.jpg|thumb|Magnetic mirrors suffered from end losses, requiring high power, complex magnetic designs, such as the baseball coil pictured here.]] |
|||
{{multiple image |
|||
| image1 = Novette laser.jpg |
|||
| width1 = 150 |
|||
| caption1 = The Novette target chamber (metal sphere with diagnostic devices protruding radially), which was reused from the [[shiva laser|Shiva]] project and two newly built laser chains visible in background |
|||
| image2 = Fusion target implosion on NOVA laser.jpg |
|||
| width2 = 150 |
|||
| caption2 = Inertial confinement fusion implosion on the [[Nova laser]] during the 1980s was a key driver of fusion development. |
|||
}} |
|||
=== First inertial confinement experiments === |
|||
Some problems which are expected to be an issue in this century such as [[water resources|fresh water shortages]] can alternatively be regarded as problems of energy supply. For example, in [[desalination]] plants, [[seawater]] can be purified through [[distillation]] or [[reverse osmosis]]. However, these processes are energy intensive. Even if the first fusion plants are not competitive with alternative sources, fusion could still become competitive if large scale desalination requires more power than the alternatives are able to provide. |
|||
{{multiple image |
|||
| total_width = 440 |
|||
| image1 = Shiva amplifier chains.jpg |
|||
| caption1 = Shiva laser, 1977, the largest ICF laser system built in the seventies |
|||
| image2 = The Tandem Mirror Experiment.jpg |
|||
| caption2 = The Tandem Mirror Experiment (TMX) in 1979 }} |
|||
Laser fusion was suggested in 1962 by scientists at [[Lawrence Livermore National Laboratory]] (LLNL), shortly after the invention of the laser in 1960. [[Inertial confinement fusion]] (using lasers) research began as early as 1965. Several laser systems were built at LLNL. These included the [[Argus laser|Argus]], the [[Cyclops laser|Cyclops]], the [[Janus laser|Janus]], the [[Long path laser|long path]], the [[Shiva laser]], and the [[Nova (laser)|Nova]].<ref>{{cite journal | doi = 10.1088/0029-5515/25/9/063 | volume=25 | title=Highlights of laser fusion related research by United Kingdom universities using the SERC Central Laser Facility at the Rutherford Appleton Laboratory | year=1985 | journal=Nuclear Fusion | pages=1351–1353 | last1 = Key | first1 = M.H.| issue=9 | s2cid=119922168 }}</ref> |
|||
A scenario has been presented of the effect of the commercialization of fusion power on the future of human civilization.<ref>{{Cite web |
|||
|url=http://www.plasmafocus.net/IPFS/2010%20Papers/LSmankind.pdf|title=Nuclear Fusion Energy-Mankind's Giant Step Forward|author=Sing Lee and Sor Heoh Saw}}</ref> ITER and later Demo are envisioned to bring online the first commercial nuclear fusion energy reactor by 2050. Using this as the starting point and the history of the uptake of nuclear fission reactors as a guide, the scenario depicts a rapid take up of nuclear fusion energy starting after the middle of this century. |
|||
Laser advances included frequency-tripling crystals that transformed infrared laser beams into ultraviolet beams and "chirping", which changed a single wavelength into a full spectrum that could be amplified and then reconstituted into one frequency.<ref>{{Cite book |title=Inertial confinement nuclear fusion : a historical approach by its pioneers|date=2007|publisher=Foxwell & Davies (UK)|editor=Verlarde, G. |editor2=Carpintero–Santamaría, Natividad |isbn=978-1905868100|location=London |oclc=153575814}}</ref> Laser research ate money as well, consuming over one billion dollars in the 1980s.<ref name="NRDC">{{cite web |last1=McKinzie |first1=Matthew |last2=Paine |first2=Christopher E. |date=2000 |title=When peer review fails: The Roots of the National Ignition Facility (NIF) Debacle |url=http://www.nrdc.org/nuclear/nif2/findings.asp |access-date=30 October 2014 |publisher=National Resources Defense Council}}</ref> |
|||
==Current status== |
|||
Despite optimism dating back to the 1950s about the wide-scale harnessing of fusion power, there are still significant barriers standing between current scientific understanding and technological capabilities and the practical realization of fusion as an energy source. Research, while making steady progress, has also continually thrown up new difficulties. Therefore it remains unclear whether an economically viable fusion plant is possible.<ref>[http://www.scientificamerican.com/article.cfm?id=fusions-false-dawn Fusion’s False Dawn] by Michael Moyer ''[[Scientific American]]'' March 2010</ref><ref name="newscientist">{{Cite web|url=http://www.newscientist.com/channel/opinion/mg19025543.300-editorial-nuclear-fusion-must-be-worth-the-gamble.html |title=Editorial: Nuclear fusion must be worth the gamble |publisher=''[[New Scientist]]'' |date=7 June 2006}}</ref> A 2006 editorial in ''[[New Scientist]]'' magazine opined that "if commercial fusion is viable, it may well be a century away."<ref name="newscientist" /> Interestingly, a pamphlet printed by [[General Atomics]] in 1970s stated that "By the year 2000, several commercial fusion reactors are expected to be on-line." |
|||
=== 1980s === |
|||
Several fusion D-T burning tokamak test devices have been built (TFTR, JET), but these were not built to produce more thermal energy than electrical energy consumed. The [[ITER]] project is currently leading the effort to commercialize fusion power.{{Citation needed|date=January 2011}} |
|||
The [[Tore Supra]], [[Joint European Torus|JET]], [[T-15 (reactor)|T-15]], and [[JT-60]] tokamaks were built in the 1980s.<ref>{{cite web|url=http://www-drfc.cea.fr/gb/cea/ts/ts.htm |title=Tore Supra |access-date=February 3, 2016 |url-status=dead |archive-url=https://web.archive.org/web/20121115112229/http://www-drfc.cea.fr/gb/cea/ts/ts.htm |archive-date=November 15, 2012 }}</ref><ref>{{Cite journal |last=Smirnov |first=V. P. |date=2009-12-30 |title=Tokamak foundation in USSR/Russia 1950–1990 |url=http://fire.pppl.gov/nf_50th_5_Smirnov.pdf |journal=Nuclear Fusion |volume=50 |issue=1 |pages=014003 |doi=10.1088/0029-5515/50/1/014003 |issn=0029-5515 |s2cid=17487157}}</ref> In 1984, Martin Peng of ORNL proposed the [[spherical tokamak]] with a much smaller radius.<ref>Y-K Martin Peng, "Spherical Torus, Compact Fusion at Low Yield". Oak Ridge National Laboratory/FEDC-87/7 (December 1984)</ref> It used a single large conductor in the center, with magnets as half-rings off of this conductor. The aspect ratio fell to as low as 1.2.<ref name="Small Tight">{{Cite journal|last=Sykes|first=Alan|date=1997|title=High β produced by neutral beam injection in the START (Small Tight Aspect Ratio Tokamak) spherical tokamak|journal=Physics of Plasmas|language=en|volume=4|issue=5|pages=1665–1671|doi=10.1063/1.872271|bibcode=1997PhPl....4.1665S|issn=1070-664X|doi-access=free}}</ref><sup>:B247</sup><ref>{{Cite book|last=Braams, C. M. |title=Nuclear fusion: half a century of magnetic confinement fusion research|author2=Stott, P. E. |year=2002|publisher=Institute of Physics Pub. |isbn=978-0367801519|oclc=1107880260}}</ref><sup>:225</sup> Peng's advocacy caught the interest of [[Derek Robinson (physicist)|Derek Robinson]], who built the [[Small Tight Aspect Ratio Tokamak]], (START).<ref name="Small Tight" /> |
|||
=== 1990s === |
|||
A paper published in January 2009 and part of the IAEA Fusion Conference Proceedings at Geneva last October claims that small 50 MW Tokamak style reactors are feasible.<ref>[http://www.claverton-energy.com/physics-and-engineering-basis-of-multi-functional-compact-tokamak.html physics and engineering basis of multi-functional compact tokamak ]</ref> |
|||
<!-- {{multiple image |
|||
| width1 = 220 |
|||
| image1 = [[WP:NFCC]] violation: Z-machine480.jpg |
|||
| caption1 = Z Machine (a pinch at SNL) went through a number of upgrades during the mid to late nineties }} --> |
|||
In 1991, the Preliminary Tritium Experiment at the [[Joint European Torus]] achieved the world's first controlled release of fusion power.<ref>{{Cite journal |last1=Jarvis |first1=O. N. |date=2006-06-16 |title=Neutron measurements from the preliminary tritium experiment at JET (invited) |journal=Review of Scientific Instruments |volume=63 |issue=10 |pages=4511–4516 |doi=10.1063/1.1143707}}</ref> |
|||
On May 30, 2009, the US [[Lawrence Livermore National Laboratory]] (LLNL), primarily a weapons lab, announced the creation of a high-energy laser system, the [[National Ignition Facility]], which can heat hydrogen atoms to temperatures only existing in nature in the cores of stars. The new laser is expected to have the ability to produce, for the first time, more energy from controlled, inertially confined nuclear fusion than was required to initiate the reaction.<ref>[http://www.breitbart.com/article.php?id=CNG.12fab6f6c00a65e15e6fb5e305aacbb7.41&show_article=1 "US lab debuts super laser", Breitbart news site]</ref> |
|||
In 1996, Tore Supra created a plasma for two minutes with a current of almost 1 million amperes, totaling 280 MJ of injected and extracted energy.<ref>{{Cite journal|last=Garin|first=Pascal|date=October 2001|title=Actively cooled plasma facing components in Tore Supra|url=http://dx.doi.org/10.1016/s0920-3796(01)00242-3|journal=Fusion Engineering and Design|volume=56–57|pages=117–123|doi=10.1016/s0920-3796(01)00242-3|bibcode=2001FusED..56..117G |issn=0920-3796}}</ref> |
|||
On January 28, 2010, the LLNL announced tests using all 192 laser beams, although with lower laser energies, smaller [[hohlraum]] targets, and substitutes for the fusion fuel capsules.<ref>{{Cite news|url=http://news.bbc.co.uk/2/hi/science/nature/8485669.stm|title=Laser fusion test results raise energy hopes|publisher=BBC News|accessdate=2010-01-29 | date=January 28, 2010}}</ref><ref>{{Cite web|url=https://publicaffairs.llnl.gov/news/news_releases/2010/NR-10-01-06.html|title=Initial NIF experiments meet requirements for fusion ignition|publisher=Lawrence Livermore National Laboratory|accessdate=2010-01-29}}</ref> More than one megajoule of ultraviolet energy was fired into the hohlraum, besting the previous world record by a factor of more than 30. The results gave the scientists confidence that they will be able to achieve ignition in more realistic tests scheduled to begin in the summer of 2010.<ref>[http://news.bbc.co.uk/2/hi/science/nature/8485669.stm "BBC:Laser fusion test results raise energy hopes"]</ref> |
|||
In 1997, JET produced a peak of 16.1 MW of fusion power (65% of heat to plasma<ref>{{Cite book |author=European Commission Directorate-General for Research and Innovation |year=2004 |title=Fusion Research: An Energy Option for Europe's Future |location=Luxembourg |publisher=Office for Official Publications of the European Communities |isbn=92-894-7714-8 |oclc=450075815}}</ref>), with fusion power of over 10 MW sustained for over 0.5 sec.<ref>{{Cite book |last=Claessens |first=Michel|date=2020|title=ITER: The Giant Fusion Reactor|url=http://dx.doi.org/10.1007/978-3-030-27581-5|doi=10.1007/978-3-030-27581-5|isbn=978-3030275808|s2cid=243590344}}</ref> |
|||
NIF researchers are currently conducting a series of "tuning" shots to determine the optimal target design and laser parameters for high-energy ignition experiments with fusion fuel in the coming months. Two firing tests have been performed on October 31 and November 2.<ref>https://www.llnl.gov/news/newsreleases/2010/Nov/NR-10-11-02.html</ref> |
|||
=== 2000s === |
|||
[[File:MAST plasma image.jpg|thumbnail|The [[Mega Ampere Spherical Tokamak]] became operational in the UK in 1999.]] |
|||
"Fast ignition"<ref>{{Cite book |last=Atzeni |first=Stefano |title=The physics of inertial fusion : beam plasma interaction, hydrodynamics, hot dense matter|date=2004|publisher=Clarendon Press|others=Meyer-ter-Vehn, Jürgen|isbn=978-0198562641|location=Oxford|oclc=56645784}}</ref><ref>{{Cite book|last=Pfalzner|first=Susanne|url=http://dx.doi.org/10.1201/9781420011845|title=An Introduction to Inertial Confinement Fusion|date=2006-03-02|publisher=CRC Press|doi=10.1201/9781420011845|isbn=978-0429148156}}</ref> saved power and moved ICF into the race for energy production. |
|||
In 2006, China's [[Experimental Advanced Superconducting Tokamak]] (EAST) test reactor was completed.<ref>{{Cite web|title=People's Daily Online – China to build world's first "artificial sun" experimental device|url=http://en.people.cn/200601/21/eng20060121_237208.html|access-date=2020-10-10|website=en.people.cn|archive-date=2011-06-05 |archive-url=https://web.archive.org/web/20110605190505/http://english.people.com.cn/200601/21/eng20060121_237208.html|url-status=dead}}</ref> It was the first tokamak to use superconducting magnets to generate both toroidal and poloidal fields. |
|||
In March 2009, the laser-driven ICF [[National Ignition Facility|NIF]] became operational.<ref>{{Cite web |title=What Is the National Ignition Facility? |url=https://lasers.llnl.gov/about/what-is-nif |access-date=2022-08-07 |website=lasers.llnl.gov |archive-url=https://web.archive.org/web/20170731064919/https://lasers.llnl.gov/about/what-is-nif |archive-date=July 31, 2017 |publisher=Lawrence Livermore National Laboratory}}</ref> |
|||
In the 2000s, privately backed fusion companies entered the race, including [[TAE Technologies]],<ref>{{Cite news|url=https://www.forbes.com/sites/michaelkanellos/2013/03/11/hollywood-silicon-valley-and-russia-join-forces-on-nuclear-fusion/#12c2795972ba|title=Hollywood, Silicon Valley and Russia Join Forces on Nuclear Fusion|last=Kanellos|first=Michael|work=Forbes|access-date=2017-08-21|language=en}}</ref> [[General Fusion]],<ref>{{Cite web|last=Frochtzwajg|first=Jonathan|title=The secretive, billionaire-backed plans to harness fusion|url=http://www.bbc.com/future/story/20160428-the-secretive-billionaire-backed-plans-to-harness-fusion|access-date=2017-08-21|website=BBC}}</ref><ref>{{Cite journal|last=Clery|first=Daniel|date=2014-07-25|title=Fusion's restless pioneers|journal=Science|language=en|volume=345|issue=6195|pages=370–375|bibcode=2014Sci...345..370C|doi=10.1126/science.345.6195.370|issn=0036-8075|pmid=25061186|ref=none}}</ref> and [[Tokamak Energy]].<ref>{{Cite web |last=Gray |first=Richard |date=19 April 2017 |title=The British reality TV star building a fusion reactor |url=http://www.bbc.com/future/story/20170418-the-made-in-chelsea-star-building-a-fusion-reactor |access-date=2017-08-21 |website=BBC}}</ref> |
|||
=== 2010s === |
|||
[[File:Preamplifier at the National Ignition Facility.jpg|upright=1.15|thumb|The preamplifiers of the National Ignition Facility. In 2012, the NIF achieved a 500-terawatt shot.]] |
|||
[[File:Wendelstein7-X Torushall-2011.jpg|upright=1.15|thumb|The Wendelstein7X under construction]] |
|||
[[File:W7X-Spulen Plasma blau gelb.jpg|thumb|upright=1.15|Example of a stellarator design: A coil system (blue) surrounds plasma (yellow). A magnetic field line is highlighted in green on the yellow plasma surface.]] |
|||
Private and public research accelerated in the 2010s. General Fusion developed plasma injector technology and Tri Alpha Energy tested its C-2U device.<ref>{{Cite journal|last=Clery|first=Daniel|date=2017-04-28|title=Private fusion machines aim to beat massive global effort|journal=Science|language=en|volume=356|issue=6336|pages=360–361|bibcode=2017Sci...356..360C|doi=10.1126/science.356.6336.360|issn=0036-8075|pmid=28450588|s2cid=206621512}}</ref> The French [[Laser Mégajoule]] began operation. NIF achieved net energy gain<ref>{{cite web |title=PW 2012: fusion laser on track for 2012 burn |publisher=Optics.org |author=SPIE Europe Ltd |url=http://optics.org/news/3/1/37 |access-date=2013-06-22}}</ref> in 2013, as defined in the very limited sense as the hot spot at the core of the collapsed target, rather than the whole target.<ref>{{cite news |url=https://www.bbc.co.uk/news/science-environment-24429621 |title=Nuclear fusion milestone passed at US lab |publisher=BBC News |access-date=30 October 2014}}</ref> |
|||
In 2014, [[Phoenix Nuclear Labs]] sold a high-yield [[neutron generator]] that could sustain 5×10<sup>11</sup> [[deuterium]] fusion reactions per second over a 24-hour period.<ref>{{cite web|url=http://phoenixnuclearlabs.com/product/high-yield-neutron-generator/|title=The Alectryon High Yield Neutron Generator|year=2013|publisher=Phoenix Nuclear Labs}}</ref> |
|||
In 2015, [[MIT]] announced a [[tokamak]] it named the [[ARC fusion reactor]], using [[rare-earth barium-copper oxide]] (REBCO) superconducting tapes to produce high-magnetic field coils that it claimed could produce comparable magnetic field strength in a smaller configuration than other designs.<ref>{{cite news |last=Chandler |first=David L. |title=A small, modular, efficient fusion plant |work=MIT News |publisher=MIT News Office |url=http://newsoffice.mit.edu/2015/small-modular-efficient-fusion-plant-0810 |date=10 August 2015}}</ref> |
|||
In October, researchers at the [[Max Planck Institute of Plasma Physics]] in Greifswald, Germany, completed building the largest [[stellarator]] to date, the [[Wendelstein 7-X]] (W7-X). The W7-X stellarator began Operational phase 1 (OP1.1) on 10 December 2015, successfully producing helium plasma.<ref>{{Cite journal|url=https://iopscience.iop.org/article/10.1088/0029-5515/55/12/126001|title=Plans for the first plasma operation of Wendelstein 7-X|journal=Nuclear Fusion |date=November 2015 |volume=55 |issue=12 |page=126001 |doi=10.1088/0029-5515/55/12/126001 |last1=Sunn Pedersen |first1=T. |last2=Andreeva |first2=T. |last3=Bosch |first3=H. -S |last4=Bozhenkov |first4=S. |last5=Effenberg |first5=F. |last6=Endler |first6=M. |last7=Feng |first7=Y. |last8=Gates |first8=D. A. |last9=Geiger |first9=J. |last10=Hartmann |first10=D. |last11=Hölbe |first11=H. |last12=Jakubowski |first12=M. |last13=König |first13=R. |last14=Laqua |first14=H. P. |last15=Lazerson |first15=S. |last16=Otte |first16=M. |last17=Preynas |first17=M. |last18=Schmitz |first18=O. |last19=Stange |first19=T. |last20=Turkin |first20=Y. |bibcode=2015NucFu..55l6001P |hdl=11858/00-001M-0000-0029-04EB-D |s2cid=67798335 |hdl-access=free }}</ref> The objective was to test vital systems and understand the machine's physics. By February 2016, hydrogen plasma was achieved, with temperatures reaching up to 100 million Kelvin. The initial tests used five graphite limiters. After over 2,000 pulses and achieving significant milestones, OP1.1 concluded on 10 March 2016. An upgrade followed, and OP1.2 in 2017 aimed to test an uncooled divertor. By June 2018, record temperatures were reached. W7-X concluded its first campaigns with limiter and island divertor tests, achieving notable advancements by the end of 2018.<ref>{{cite journal |title=Confirmation of the topology of the Wendelstein 7-X magnetic field to better than 1:100,000 |journal=Nature Communications |volume=7 |pages=13493 |doi=10.1038/ncomms13493|pmid=27901043 |pmc=5141350 |year=2016 |last1=Pedersen |first1=T. Sunn |last2=Otte |first2=M. |last3=Lazerson |first3=S. |last4=Helander |first4=P. |last5=Bozhenkov |first5=S. |last6=Biedermann |first6=C. |last7=Klinger |first7=T. |last8=Wolf |first8=R. C. |last9=Bosch |first9=H. -S. |last10=Abramovic |first10=Ivana |last11=Äkäslompolo |first11=Simppa |last12=Aleynikov |first12=Pavel |last13=Aleynikova |first13=Ksenia |last14=Ali |first14=Adnan |last15=Alonso |first15=Arturo |last16=Anda |first16=Gabor |last17=Andreeva |first17=Tamara |last18=Ascasibar |first18=Enrique |last19=Baldzuhn |first19=Jürgen |last20=Banduch |first20=Martin |last21=Barbui |first21=Tullio |last22=Beidler |first22=Craig |last23=Benndorf |first23=Andree |last24=Beurskens |first24=Marc |last25=Biel |first25=Wolfgang |last26=Birus |first26=Dietrich |last27=Blackwell |first27=Boyd |last28=Blanco |first28=Emilio |last29=Blatzheim |first29=Marko |last30=Bluhm |first30=Torsten |display-authors=29 |bibcode=2016NatCo...713493P }}</ref><ref>{{Cite journal|title=Performance of Wendelstein 7-X stellarator plasmas during the first divertor operation phase|first1=R. C.|last1=Wolf|first2=A.|last2=Alonso|first3=S.|last3=Äkäslompolo|first4=J.|last4=Baldzuhn|first5=M.|last5=Beurskens|first6=C. D.|last6=Beidler|first7=C.|last7=Biedermann|first8=H.-S.|last8=Bosch|first9=S.|last9=Bozhenkov|first10=R.|last10=Brakel|first11=H.|last11=Braune|first12=S.|last12=Brezinsek|first13=K.-J.|last13=Brunner|first14=H.|last14=Damm|first15=A.|last15=Dinklage|first16=P.|last16=Drewelow|first17=F.|last17=Effenberg|first18=Y.|last18=Feng|first19=O.|last19=Ford|first20=G.|last20=Fuchert|first21=Y.|last21=Gao|first22=J.|last22=Geiger|first23=O.|last23=Grulke|first24=N.|last24=Harder|first25=D.|last25=Hartmann|first26=P.|last26=Helander|first27=B.|last27=Heinemann|first28=M.|last28=Hirsch|first29=U.|last29=Höfel|first30=C.|last30=Hopf|first31=K.|last31=Ida|first32=M.|last32=Isobe|first33=M. W.|last33=Jakubowski|first34=Y. O.|last34=Kazakov|first35=C.|last35=Killer|first36=T.|last36=Klinger|first37=J.|last37=Knauer|first38=R.|last38=König|first39=M.|last39=Krychowiak|first40=A.|last40=Langenberg|first41=H. P.|last41=Laqua|first42=S.|last42=Lazerson|first43=P.|last43=McNeely|first44=S.|last44=Marsen|first45=N.|last45=Marushchenko|first46=R.|last46=Nocentini|first47=K.|last47=Ogawa|first48=G.|last48=Orozco|first49=M.|last49=Osakabe|first50=M.|last50=Otte|first51=N.|last51=Pablant|first52=E.|last52=Pasch|first53=A.|last53=Pavone|first54=M.|last54=Porkolab|first55=A.|last55=Puig Sitjes|first56=K.|last56=Rahbarnia|first57=R.|last57=Riedl|first58=N.|last58=Rust|first59=E.|last59=Scott|first60=J.|last60=Schilling|first61=R.|last61=Schroeder|first62=T.|last62=Stange|first63=A.|last63=von Stechow|first64=E.|last64=Strumberger|first65=T.|last65=Sunn Pedersen|first66=J.|last66=Svensson|first67=H.|last67=Thomson|first68=Y.|last68=Turkin|first69=L.|last69=Vano|first70=T.|last70=Wauters|first71=G.|last71=Wurden|first72=M.|last72=Yoshinuma|first73=M.|last73=Zanini|first74=D.|last74=Zhang|date=1 August 2019|journal=Physics of Plasmas|volume=26|issue=8|pages=082504|doi=10.1063/1.5098761|bibcode=2019PhPl...26h2504W |s2cid=202127809 |doi-access=free|hdl=1721.1/130063|hdl-access=free}}</ref><ref>{{Cite journal|title=Experimental confirmation of efficient island divertor operation and successful neoclassical transport optimization in Wendelstein 7-X|journal=Nuclear Fusion |date=April 2022 |volume=62 |issue=4 |page=042022 |doi=10.1088/1741-4326/ac2cf5 |last1=Sunn Pedersen |first1=Thomas |last2=Abramovic |first2=I. |last3=Agostinetti |first3=P. |last4=Agredano Torres |first4=M. |last5=Äkäslompolo |first5=S. |last6=Alcuson Belloso |first6=J. |last7=Aleynikov |first7=P. |last8=Aleynikova |first8=K. |last9=Alhashimi |first9=M. |last10=Ali |first10=A. |last11=Allen |first11=N. |last12=Alonso |first12=A. |last13=Anda |first13=G. |last14=Andreeva |first14=T. |last15=Angioni |first15=C. |last16=Arkhipov |first16=A. |last17=Arnold |first17=A. |last18=Asad |first18=W. |last19=Ascasibar |first19=E. |last20=Aumeunier |first20=M. -H |last21=Avramidis |first21=K. |last22=Aymerich |first22=E. |last23=Baek |first23=S. -G |last24=Bähner |first24=J. |last25=Baillod |first25=A. |last26=Balden |first26=M. |last27=Balden |first27=M. |last28=Baldzuhn |first28=J. |last29=Ballinger |first29=S. |last30=Banduch |first30=M. |bibcode=2022NucFu..62d2022S |s2cid=234338848 |display-authors=1 |doi-access=free |hdl=1721.1/147631 |hdl-access=free }}</ref> It soon produced helium and hydrogen plasmas lasting up to 30 minutes.<ref>{{Cite web|last=Max Planck Institute for Experimental Physics|date=February 3, 2016|title=Wendelstein 7-X fusion device produces its first hydrogen plasma|url=https://www.ipp.mpg.de/4010154/02_16|access-date=2021-06-15|website=www.ipp.mpg.de|language=en}}</ref> |
|||
In 2017, [[Helion Energy]]'s fifth-generation plasma machine went into operation.<ref>{{Cite web|last=Wang|first=Brian|date=August 1, 2018|title=Nuclear Fusion Updated project reviews|url=https://www.nextbigfuture.com/2018/08/nuclear-fusion-updated-project-reviews.html|access-date=2018-08-03|website=www.nextbigfuture.com|language=en-US}}</ref> The UK's Tokamak Energy's [[Tokamak Energy|ST40]] generated "first plasma".<ref>{{Cite web|url=https://www.sciencealert.com/the-uk-has-just-switch-on-its-tokamak-nuclear-fusion-reactor|title=The UK Just Switched on an Ambitious Fusion Reactor – And It Works|last=MacDonald|first=Fiona|website=ScienceAlert|date=May 2017 |language=en-gb|access-date=2019-07-03}}</ref> The next year, [[Eni]] announced a $50 million investment in [[Commonwealth Fusion Systems]], to attempt to commercialize MIT's [[ARC fusion reactor|ARC]] technology.<ref>{{cite news |url=https://www.reuters.com/article/us-nuclearpower-fusion-eni/italys-eni-defies-skeptics-may-up-stake-in-nuclear-fusion-project-idUSKBN1HK1JJ |title=Italy's Eni defies sceptics, may up stake in nuclear fusion project |date=13 April 2018|newspaper=Reuters }}</ref><ref>{{cite web |url=https://www.seeker.com/energy/mit-aims-to-harness-fusion-power-within-15-years |title=MIT Aims to Harness Fusion Power Within 15 years |date=3 April 2018}}</ref><ref>{{cite web| url=http://www.wbur.org/bostonomix/2018/03/09/mit-nuclear-fusion |title=MIT Aims To Bring Nuclear Fusion To The Market In 10 Years |date=9 March 2018}}</ref><ref>{{cite web |last=Chandler |first=David |date=9 March 2018 |title=MIT and newly formed company launch novel approach to fusion power |url=https://news.mit.edu/2018/mit-newly-formed-company-launch-novel-approach-fusion-power-0309 |website=MIT News |publisher=Massachusetts Institute of Technology}}</ref> |
|||
=== 2020s === |
|||
In January 2021, SuperOx announced the commercialization of a new [[superconducting wire]] with more than 700 A/mm<sup>2</sup> current capability.<ref>{{cite journal |last1=Molodyk |first1=A. |last2=Samoilenkov |first2=S. |last3=Markelov |first3=A. |last4=Degtyarenko |first4=P. |last5=Lee |first5=S. |last6=Petrykin |first6=V. |last7=Gaifullin |first7=M. |last8=Mankevich |first8=A. |last9=Vavilov |first9=A. |last10=Sorbom |first10=B. |last11=Cheng |first11=J. |last12=Garberg |first12=S. |last13=Kesler |first13=L. |last14=Hartwig |first14=Z. |last15=Gavrilkin |first15=S. |last16=Tsvetkov |first16=A. |last17=Okada |first17=T. |last18=Awaji |first18=S. |last19=Abraimov |first19=D. |last20=Francis |first20=A. |last21=Bradford |first21=G. |last22=Larbalestier |first22=D. |last23=Senatore |first23=C. |last24=Bonura |first24=M. |last25=Pantoja |first25=A. E. |last26=Wimbush |first26=S. C. |last27=Strickland |first27=N. M. |last28=Vasiliev |first28=A. |title=Development and large volume production of extremely high current density YBa 2 Cu 3 O 7 superconducting wires for fusion |journal=Scientific Reports |date=22 January 2021 |volume=11 |issue=1 |pages=2084 |doi=10.1038/s41598-021-81559-z|pmid=33483553 |pmc=7822827 }}</ref> |
|||
TAE Technologies announced results for its Norman device, holding a temperature of about 60 MK for 30 milliseconds, 8 and 10 times higher, respectively, than the company's previous devices.<ref>{{Cite web|last=Clery|first=Daniel|date=2021-04-08|title=With "smoke ring" technology, fusion startup marks steady progress|url=https://www.science.org/content/article/smoke-ring-technology-fusion-startup-marks-steady-progress|access-date=2021-04-11|website=Science {{!}} AAAS|language=en}}</ref> |
|||
In October, Oxford-based [[First Light Fusion]] revealed its projectile fusion project, which fires an aluminum disc at a fusion target, accelerated by a 9 mega-amp electrical pulse, reaching speeds of {{Convert|20|km/s}}. The resulting fusion generates neutrons whose energy is captured as heat.<ref>{{Cite news|last=Morris|first=Ben|date=2021-09-30|title=Clean energy from the fastest moving objects on earth|language=en-GB |publisher=BBC News |url=https://www.bbc.com/news/business-58602159|access-date=2021-12-09}}</ref> |
|||
On November 8, in an invited talk to the 63rd Annual Meeting of the APS Division of Plasma Physics,<ref>{{Cite conference |conference=63rd Annual Meeting of the APS Division of Plasma Physics, November 8–12, 2021; Pittsburgh, PA |url=https://meetings.aps.org/Meeting/DPP21/Session/AR01?showAbstract|title = Session AR01: Review: Creating A Burning Plasma on the National Ignition Facility|volume = 66|issue = 13 |work= Bulletin of the American Physical Society}}</ref> the National Ignition Facility claimed<ref name="physics_v14_168">{{Cite journal |
|||
|url=https://physics.aps.org/articles/v14/168|title = Ignition First in a Fusion Reaction|journal = Physics|date = November 30, 2021|volume = 14|last1 = Wright|first1 = Katherine|page = 168|doi = 10.1103/Physics.14.168|bibcode = 2021PhyOJ..14..168W|s2cid = 244829710|doi-access = free}}</ref> to have triggered [[fusion ignition]] in the laboratory on August 8, 2021, for the first time in the 60+ year history of the ICF program.<ref>{{Cite web |last=Dunning |first=Hayley |date=17 August 2021 |title=Major nuclear fusion milestone reached as "ignition" triggered in a lab |url=https://phys.org/news/2021-08-major-nuclear-fusion-milestone-ignition.html |website=Science X Network}}</ref><ref>{{Cite web |last=Bishop |first=Breanna |date=18 August 2021 |title=National Ignition Facility experiment puts researchers at threshold of fusion ignition |url=https://www.llnl.gov/news/national-ignition-facility-experiment-puts-researchers-threshold-fusion-ignition |website=Lawrence Livermore National Laboratory}}</ref> The shot yielded 1.3 MJ of fusion energy, an over 8X improvement on tests done in spring of 2021.<ref name="physics_v14_168" /> NIF estimates that 230 kJ of energy reached the fuel capsule, which resulted in an almost 6-fold energy output from the capsule.<ref name="physics_v14_168" /> A researcher from Imperial College London stated that the majority of the field agreed that ignition had been demonstrated.<ref name="physics_v14_168" /> |
|||
In November 2021, [[Helion Energy]] reported receiving $500 million in Series E funding for its seventh-generation Polaris device, designed to demonstrate net electricity production, with an additional $1.7 billion of commitments tied to specific milestones,<ref>{{Cite web|last=Conca|first=James|title=Helion Energy Raises $500 Million On The Fusion Power Of Stars|url=https://www.forbes.com/sites/jamesconca/2021/11/09/helion-energy-raises-500-million-on-the-fusion-power-of-stars/|access-date=2021-12-19|website=Forbes|language=en}}</ref> while Commonwealth Fusion Systems raised an additional $1.8 billion in Series B funding to construct and operate its [[SPARC (tokamak)|SPARC tokamak]], the single largest investment in any private fusion company.<ref>{{Cite news|last=Journal|first=Jennifer Hiller {{!}} Photographs by Tony Luong for The Wall Street|date=2021-12-01|title=WSJ News Exclusive {{!}} Nuclear-Fusion Startup Lands $1.8 Billion as Investors Chase Star Power|language=en-US|work=Wall Street Journal|url=https://www.wsj.com/articles/nuclear-fusion-startup-lands-1-8-billion-as-investors-chase-star-power-11638334801|access-date=2021-12-17|issn=0099-9660}}</ref> |
|||
In April 2022, First Light announced that their hypersonic projectile fusion prototype had produced neutrons compatible with fusion. Their technique electromagnetically fires projectiles at [[Mach number|Mach]] 19 at a caged fuel pellet. The deuterium fuel is compressed at Mach 204, reaching pressure levels of 100 TPa.<ref>{{Cite web |last=Blain |first=Loz |date=2022-04-06 |title=Oxford spinoff demonstrates world-first hypersonic "projectile fusion" |url=https://newatlas.com/energy/first-light-nuclear-fusion-projectile/ |access-date=2022-04-06 |website=New Atlas |language=en-US}}</ref> |
|||
On December 13, 2022, the [[US Department of Energy]] reported that researchers at the National Ignition Facility had achieved a net energy gain from a fusion reaction. The reaction of hydrogen fuel at the facility produced about 3.15 MJ of energy while consuming 2.05 MJ of input. However, while the fusion reactions may have produced more than 3 megajoules of energy—more than was delivered to the target—NIF's 192 lasers consumed 322 MJ of grid energy in the conversion process.<ref name="NYT-209221213"/><ref name="Ignition" /><ref name="WP-20221212">{{cite news |last=Osaka |first=Shannon |title=What you need to know about the U.S. fusion energy breakthrough |url=https://www.washingtonpost.com/climate-solutions/2022/12/12/nuclear-fusion-breakthrough-benefits/ |date=12 December 2022 |newspaper=[[The Washington Post]] |access-date=13 December 2022 }}</ref><ref>{{Cite web |last=Hartsfield |first=Tom |date=December 13, 2022 |title=There is no "breakthrough": NIF fusion power still consumes 130 times more energy than it creates |url=https://bigthink.com/the-future/fusion-power-nif-hype-lose-energy/ |website=Big Think}}</ref> |
|||
In May 2023, the [[United States Department of Energy]] (DOE) provided a grant of $46 million to eight companies across seven states to support fusion power plant design and research efforts. This funding, under the Milestone-Based Fusion Development Program, aligns with objectives to demonstrate pilot-scale fusion within a decade and to develop fusion as a carbon-neutral energy source by 2050. The granted companies are tasked with addressing the scientific and technical challenges to create viable fusion pilot plant designs in the next 5–10 years. The recipient firms include Commonwealth Fusion Systems, Focused Energy Inc., Princeton Stellarators Inc., Realta Fusion Inc., Tokamak Energy Inc., Type One Energy Group, Xcimer Energy Inc., and Zap Energy Inc.<ref>{{Cite news |last=Gardner |first=Timothy |date=1 June 2023 |title=US announces $46 million in funds to eight nuclear fusion companies |agency=Reuters}}</ref> |
|||
In December 2023, the largest and most advanced tokamak JT-60SA was inaugurated in [[Naka, Ibaraki|Naka]], Japan. The reactor is a joint project between Japan and the European Union. The reactor had achieved its first plasma in October 2023.<ref>{{cite web |last=Dobberstein |first=Laura |date=4 December 2023 |title=World's largest nuclear fusion reactor comes online in Japan |url=https://www.theregister.com/2023/12/04/jt_60sa_tokamak_online/ |website=The Register |publisher=Situation Publishing}}</ref> Subsequently, South Korea's fusion reactor project, the [[Korean Superconducting Tokamak Advanced Research]], successfully operated for 102 seconds in a high-containment mode (H-mode) containing high ion temperatures of more than 100 million degrees in plasma tests conducted from December 2023 to February 2024.<ref>{{Cite news |date=March 21, 2024 |title=S. Korea's artificial sun project KSTAR achieves longest operation time of 102 seconds |url=https://m.ajudaily.com/view/20240321111705997 |work=[[Aju Business Daily]]}}</ref> |
|||
== Records == |
|||
Fusion records continue to advance: |
|||
{| class="wikitable sortable" |
|||
|+Records |
|||
! Domain !! Year !! Record !! Device !! Notes |
|||
|- |
|||
| Plasma temperature|| 2012 || 1.8{{e|9}} K || Focus-Fusion 1<ref>{{cite journal |last1=Lerner |first1=Eric J. |last2=Murali |first2=S. Krupakar |last3=Shannon |first3=Derek |last4=Blake |first4=Aaron M. |last5=Van Roessel |first5=Fred |date=23 March 2012 |title=Fusion reactions from >150 keV ions in a dense plasma focus plasmoid |journal=Physics of Plasmas |volume=19 |issue=3 |pages=032704 |bibcode=2012PhPl...19c2704L |doi=10.1063/1.3694746 |s2cid=120207711}}</ref><ref>{{cite news|last=Halper|first=Mark|title=Fusion breakthrough|url=http://www.smartplanet.com/blog/intelligent-energy/fusion-breakthrough/14516|access-date=1 April 2012|newspaper=Smart PLanet|date=March 28, 2012}}</ref> || |
|||
|- |
|||
| Fusion power || 1997 || 1.6{{e|7}} W || [[Joint European Torus|JET]]<ref name="ccfe">{{cite web|title=JET|url=http://www.ccfe.ac.uk/jet.aspx|url-status=dead|archive-url=https://web.archive.org/web/20160707193828/http://www.ccfe.ac.uk/jet.aspx|archive-date=July 7, 2016|access-date=26 June 2016|publisher=Culham Centre Fusion Energy}}</ref> || |
|||
|- |
|||
|Tokamak fusion energy |
|||
|2023 |
|||
|6.9{{e|7}} J |
|||
|JET<ref>{{Cite web |last=Tischler |first=Karl |date=2024-02-08 |title=Breaking New Ground: JET Tokamak's Latest Fusion Energy Record Shows Mastery of Fusion Processes |url=https://euro-fusion.org/eurofusion-news/dte3record/ |access-date=2024-02-11 |website=EUROfusion |language=en-US}}</ref> |
|||
| |
|||
|- |
|||
| ICF fusion energy || 2022 || 3.15{{e|6}} J || [[National Ignition Facility|NIF]]<ref name="WP-20221212"/> ||Delivering 2.05 megajoules (MJ) of light energy to the target, resulting in 3.15 MJ of fusion energy output from appr. 400 MJ electric energy to drive the lasers. |
|||
|- |
|||
| ICF shot rate || 2013 || Over 90K shots over 10 hours; Over 11 million pulse power shots at 10 Hz for over 12 days; || Electra laser at the [[Naval Research Laboratory]]<ref>{{cite journal|author=Obenschain, Stephen | display-authors=etal|title=High-energy krypton fluoride lasers for inertial fusion|journal=Applied Optics|volume=54|issue=31|year=2015|pages=F103–F122|doi=10.1364/AO.54.00F103| pmid=26560597| bibcode=2015ApOpt..54F.103O}}</ref> <ref>"Krypton Fluoride (KrF) Laser Driver for Inertial Fusion Energy"</ref> || |
|||
|- |
|||
| Plasma pressure || 2016 || 2.1{{e|5}} Pa || [[Alcator C-Mod]]<ref>{{Cite web|title=New record for fusion|url=https://news.mit.edu/2016/alcator-c-mod-tokamak-nuclear-fusion-world-record-1014|access-date=2020-10-11|website=MIT News {{!}} Massachusetts Institute of Technology|date=October 14, 2016 |language=en}}</ref> || |
|||
|- |
|||
| [[Lawson criterion]] || 2013 || 1.53{{e|24}} eV·s/m<sup>3</sup> || [[JT-60]]<ref>{{cite web |url=http://www-jt60.naka.jaea.go.jp/english/html/exp_rep/rep36.html |title=World Highest Fusion Triple Product Marked in High-βp H-mode Plasmas |archive-url=https://web.archive.org/web/20130106002319/http://www-jt60.naka.jaea.go.jp/english/html/exp_rep/rep36.html |archive-date=2013-01-06 }}</ref><ref>{{Cite web|title=Measuring Progress in Fusion Energy: The Triple Product|url=https://www.fusionenergybase.com/article/measuring-progress-in-fusion-energy-the-triple-product/|access-date=2020-10-10|website=www.fusionenergybase.com|language=en|archive-date=2020-10-01 |archive-url=https://web.archive.org/web/20201001051629/https://www.fusionenergybase.com/article/measuring-progress-in-fusion-energy-the-triple-product/|url-status=dead}}</ref> || |
|||
|- |
|||
| [[Fusion energy gain factor]] || 2022 || 1.54 || NIF<ref name="WP-20221212"/> || |
|||
|- |
|||
| Confinement time (field reversed configuration) || 2016 || 3{{e|-1}} s || [[Princeton field-reversed configuration|Princeton Field Reversed Configuration]]<ref>Cohen, Sam, and B. Berlinger. "Long-pulse Operation of the PFRC-2 Device." The Joint US-Japan Compact Torus. Wisconsin, Madison. 22 Aug. 2016. Lecture.</ref> ||Fusion was not observed. |
|||
|- |
|||
| Confinement time (stellarator) || 2019 || >1{{e|2}} s || [[Wendelstein 7-X]]<ref>{{Cite web|url=https://www.ipp.mpg.de/4550215/11_18|title=Successful second round of experiments with Wendelstein 7-X|website=www.ipp.mpg.de|language=en|access-date=22 March 2019}}</ref><ref>{{Cite web|url=https://newatlas.com/wendelstein-7-x-nuclear-fusion-records/57394|title=Wendelstein 7-X fusion reactor keeps its cool en route to record-breaking results|last=Lavars|first=Nick|date=26 November 2018|website=newatlas.com|language=en|access-date=1 December 2018}}</ref> || |
|||
|- |
|||
|Confinement time (tokamak) |
|||
|2022 |
|||
|>1{{e|3}} s |
|||
|EAST<ref>{{Cite web|url=https://www.smithsonianmag.com/smart-news/chinas-artificial-sun-reactor-broke-record-for-nuclear-fusion-180979336/|title=China's Artificial Sun Just Broke a Record for Longest Sustained Nuclear Fusion|first1=Smithsonian|last1=Magazine|first2=Elizabeth|last2=Gamillo|website=Smithsonian Magazine}}</ref> |
|||
| |
|||
|- |
|||
|Confinement time x temperature (tokamak) |
|||
|2021 |
|||
|1.2{{e|10}} K·s |
|||
|EAST<ref>{{Cite web|title=China's "Artificial Sun" Fusion Reactor Just Set a World Record|url=https://futurism.com/chinas-artificial-sun-fusion-reactor-just-set-a-world-record|website=Futurism|date=June 2, 2021 }}</ref> |
|||
| |
|||
|- |
|||
| [[Plasma beta|Beta]] || 1998 || 0.4 || [[Small Tight Aspect Ratio Tokamak]]<ref>Alan Sykes, [http://www.triam.kyushu-u.ac.jp/ICPP/program/download/12-PL01.pdf "The Development of the Spherical Tokamak"] {{webarchive |url=https://web.archive.org/web/20110722072454/http://www.triam.kyushu-u.ac.jp/ICPP/program/download/12-PL01.pdf |date=July 22, 2011 }}, ICPP, Fukuoka September 2008</ref> || |
|||
|- |
|||
|Temperature (compact spherical tokamak) |
|||
|2022 |
|||
|1{{e|8}} K |
|||
|[[Tokamak Energy]]<ref>{{Cite web |last=Szondy |first=David |date=2022-03-13 |title=Tokamak Energy achieves temperature threshold for commercial fusion |url=https://newatlas.com/energy/tokamak-energy-temperature-threshold-commercial-fusion/ |access-date=2022-03-15 |website=New Atlas |language=en-US}}</ref> |
|||
| |
|||
|- |
|||
|Temperature x time (tokamak) |
|||
|2021 |
|||
|3{{e|9}} K·s |
|||
|[[KSTAR]]<ref>{{Cite web |last=Lavars |first=Nick |date=2021-11-24 |title=KSTAR fusion reactor sets record with 30-second plasma confinement |url=https://newatlas.com/energy/kstar-fusion-reactor-record-30-second-plasma/ |access-date=2022-03-15 |website=New Atlas |language=en-US}}</ref> |
|||
| |
|||
|} |
|||
<!-- |
|||
Fusion power trends as the plasma confinement raised to the fourth power.<ref>"Fusion energy and why it is important to chase the impossible" Dr. Melanie Windridge, TED x Warwick, April 19th 2018.</ref> Hence, getting a strong plasma trap is of real value to a fusion power plant. Plasma has a very good [[electrical conductivity]]. This opens the possibility of confining the plasma with [[magnetic field]], generally known as [[magnetic confinement fusion|magnetic confinement]]. The field puts a magnetic pressure on the plasma, which holds it in. A widely used measure of magnetic trapping in fusion is the beta ratio (plasma pressure/magnetic field pressure): |
|||
<math>\beta = \frac{p}{p_{mag}} = \frac{n k_B T}{(B^2/2\mu_0)}</math><ref>{{Cite book|last=Wesson, John |title=Tokamaks|date=2004|publisher=Clarendon Press|others=Campbell, D. J.|isbn=0198509227|edition=3rd|location=Oxford|oclc=52324306}}</ref>{{rp|115}} |
|||
This is the ratio of the externally applied field to the internal pressure of the plasma. A value of 1 is ideal trapping. Some examples of beta values include: |
|||
# The [[Small Tight Aspect Ratio Tokamak|START]] machine: 0.32 |
|||
# The [[Levitated dipole]] experiment:<ref>{{Cite journal|title=APS – 50th Annual Meeting of the Division of Plasma Physics – Event – Improved Confinement During Magnetic Levitation in LDX|url=https://meetings.aps.org/Meeting/DPP08/Session/CI1.6|journal=Bulletin of the American Physical Society|publisher=American Physical Society|volume=53|issue=14}}</ref> 0.26 |
|||
# Spheromaks: ≈ 0.1,<ref name = "WHAT">{{cite journal | last1 = Ono | first1 = Y | year = 1999 | title = New relaxation of merging spheromaks to a field reversed configuration | journal = Nuclear Fusion | volume = 39 | issue = 11Y| pages = 2001–2008 | doi = 10.1088/0029-5515/39/11Y/346 | bibcode = 1999NucFu..39.2001O }}</ref> Maximum 0.2 based on Mercier limit.<ref>{{Cite web|last1=Fowler|first1=T. K.|last2=Hooper|first2=E. B.|date=1996-06-19|title=Advanced spheromak fusion reactor|url=https://digital.library.unt.edu/ark:/67531/metadc682204/|access-date=2020-10-11|website=ICENES `96: emerging nuclear energy systems, Obninsk (Russian Federation), Jun 1996|language=en}}</ref> |
|||
# The [[DIII-D]] machine: 0.126 {{Citation needed|date=March 2015}} |
|||
# The [[Gas Dynamic Trap]] a magnetic mirror: 0.6<ref>{{cite journal|title=Three Game Changing Discoveries: A Simpler Fusion Concept?|first=Thomas C.|last=Simonen|s2cid=122088138|journal=Journal of Fusion Energy|date=2016|volume=35|pages=63–68|doi=10.1007/s10894-015-0017-2}}</ref> for 5E−3 seconds.<ref name="Present">Gas Dynamic Trap (GDT). Experiments with Electron Heating. Budker Institute of Nuclear Physics, Novosibirsk State University. Siberian Branch, Russia, 2012, Thomas Simonen</ref> |
|||
# The Sustained Spheromak Plasma Experiment at Los Alamos National labs < 0.05 for 4E−6 seconds.<ref>{{Cite journal|last1=Wood|first1=R.D.|last2=Hill|first2=D.N.|last3=McLean|first3=H.S.|last4=Hooper|first4=E. B.|last5=Hudson|first5=B.F.|last6=Moller|first6=J.M.|last7=Romero-Talamás|first7=C.A.|date=2008-12-30|title=Improved magnetic field generation efficiency and higher temperature spheromak plasmas|url=http://dx.doi.org/10.1088/0029-5515/49/2/025001|journal=Nuclear Fusion|volume=49|issue=2|pages=025001|doi=10.1088/0029-5515/49/2/025001|osti=947748|issn=0029-5515}}</ref> |
|||
--> |
|||
== See also == |
== See also == |
||
{{Portal |
{{Portal|Energy|Nuclear technology}} |
||
{{cols}} |
|||
*[[List of fusion experiments]] |
|||
* [[COLEX process]], for production of Li-6 |
|||
*[[Nuclear fusion]] |
|||
*[[ |
* [[Fusion ignition]] |
||
* [[High beta fusion reactor]] |
|||
* [[Inertial electrostatic confinement]] |
|||
* [[Levitated dipole]] |
|||
* [[List of fusion experiments]] |
|||
* [[Magnetic mirror]] |
|||
{{colend}} |
|||
== References == |
|||
{{Reflist|refs= |
|||
<ref name="ProliferationRisk_Goldston">R. J. Goldston, A. Glaser, A. F. Ross: [http://web.mit.edu/fusion-fission/HybridsPubli/Fusion_Proliferation_Risks.pdf "Proliferation Risks of Fusion Energy: Clandestine Production, Covert Production, and Breakout"] {{Webarchive|url=https://web.archive.org/web/20140227005532/http://web.mit.edu/fusion-fission/HybridsPubli/Fusion_Proliferation_Risks.pdf |date=2014-02-27 }};''9th IAEA Technical Meeting on Fusion Power Plant Safety'' (accessible at no cost, 2013) and {{Cite journal|last1=Glaser|first1=A.|last2=Goldston|first2=R. J.|doi= 10.1088/0029-5515/52/4/043004|title= Proliferation risks of magnetic fusion energy: Clandestine production, covert production and breakout|journal=Nuclear Fusion|volume=52|issue=4|at=043004|year=2012|bibcode= 2012NucFu..52d3004G|s2cid=73700489 }}</ref> |
|||
<ref name="StrongNeutronSources">{{cite conference|url= http://www.ianus.tu-darmstadt.de/media/ianus/pdfs/matthias/Strong_Neutron_Sources_final.pdf|title=Strong Neutron Sources – How to cope with weapon material production capabilities of fusion and spallation neutron sources?|url-status=dead|archive-url= https://web.archive.org/web/20140224024536/http://www.ianus.tu-darmstadt.de/media/ianus/pdfs/matthias/Strong_Neutron_Sources_final.pdf|archive-date=2014-02-24|first1=Matthias|last1=Englert|first2=Giorgio|last2=Franceschini|first3=Wolfgang|last3=Liebert|date=2011|conference=7th INMM/Esarda Workshop, Aix-en-Provence}}</ref> |
|||
}} |
|||
== Bibliography == |
|||
* {{cite book|first=Daniel |last=Clery|title=A Piece of the Sun: The Quest for Fusion Energy|url={{google books |plainurl=y |id=EGcjCQAAQBAJ&|page=1}}|date=2014|publisher=The Overlook Press|isbn=978-1468310412}} |
|||
* {{cite book|last1=Cockburn |first1=Stewart |last2=Ellyard |first2=David |title=Oliphant, the life and times of Sir Mark Oliphant |date=1981 |publisher=Axiom Books |isbn=978-0959416404}} |
|||
* {{cite book|first=Stephen O. |last=Dean|title=Search for the Ultimate Energy Source: A History of the U.S. Fusion Energy Program|url={{google books |plainurl=y |id=KSA_AAAAQBAJ|page=212}}|date= 2013|publisher=Springer Science & Business Media|isbn=978-1461460374}} |
|||
* {{cite book|last1=Hagelstein |first1=Peter L. |author-link=Peter L. Hagelstein |last2=McKubre |first2=Michael |author-link2=Michael McKubre|last3=Nagel |first3=David |last4=Chubb |first4=Talbot |last5=Hekman |first5=Randall |title=11th Condensed Matter Nuclear Science |chapter=New Physical Effects in Metal Deuterides |ref={{harvid|Hagelstein et al.|2004}} |location=Washington |publisher=US Department of Energy |date=2004 |volume=11 |pages=23–59 |doi=10.1142/9789812774354_0003 |bibcode=2006cmns...11...23H |isbn=978-9812566409 |citeseerx=10.1.1.233.5518 |chapter-url=http://www.science.doe.gov/Sub/Newsroom/News_Releases/DOE-SC/2004/low_energy/Appendix_1.pdf |url-status=dead |archive-url=https://web.archive.org/web/20070106185101/http://www.science.doe.gov/Sub/Newsroom/News_Releases/DOE-SC/2004/low_energy/Appendix_1.pdf |archive-date=January 6, 2007 }} (manuscript) |
|||
* {{cite magazine|last=Hutchinson|first=Alex|title=The Year in Science: Physics|magazine=Discover Magazine (Online)|date=January 8, 2006|url=http://discovermagazine.com/2006/jan/physics|access-date=2008-06-20|issn=0274-7529}} |
|||
* Nuttall, William J., Konishi, Satoshi, Takeda, Shutaro, and Webbe-Wood, David (2020). ''[https://books.google.com/books?id=QVUvzgEACAAJ Commercialising Fusion Energy: How Small Businesses are Transforming Big Science]''. IOP Publishing. {{ISBN|978-0750327176}}. |
|||
* {{cite book|first=Andrés de Bustos |last=Molina|title=Kinetic Simulations of Ion Transport in Fusion Devices|url={{google books |plainurl=y |id=adMWmwEACAAJ}}|date= 2013|publisher=Springer International Publishing|isbn=978-3319004211}} |
|||
* {{cite book|last=Nagamine|first=Kanetada|date=2003|title=Introductory Muon Science|publisher=Cambridge University Press|chapter=Muon Catalyzed Fusion|isbn=978-0521038201}} |
|||
* {{cite book|last=Pfalzner|first=Susanne|title=An Introduction to Inertial Confinement Fusion|date=2006|publisher=Taylor & Francis|location=US|isbn=978-0750307017}} |
|||
==Further reading== |
|||
* {{Cite magazine|last=Ball|first=Philip|magazine=[[Nature (magazine)|Nature]]|title=The chase for fusion energy|url=https://www.nature.com/immersive/d41586-021-03401-w/index.html|access-date=2021-11-22 |language=en}} |
|||
* [[Naomi Oreskes|Oreskes, Naomi]], "Fusion's False Promise: Despite a recent advance, [[nuclear fusion]] is not the solution to the [[climate crisis]]", ''[[Scientific American]]'', vol. 328, no. 6 (June 2023), p. 86. |
|||
== External links == |
|||
==References== |
|||
{{commons category|Nuclear fusion reactors}} |
|||
{{No footnotes|date=October 2009}} |
|||
* [https://nucleus.iaea.org/sites/fusionportal/Pages/FusDIS.aspx Fusion Device Information System] |
|||
{{Reflist|2}} |
|||
* [https://www.fusionenergybase.com/ Fusion Energy Base] |
|||
* [https://www.fusionindustryassociation.org/ Fusion Industry Association] |
|||
* [https://www.psatellite.com/fusion-power-and-propulsion-in-the-news/ Princeton Satellite Systems News] |
|||
* [https://web.archive.org/web/20190105193140/https://science.energy.gov/fes/ U.S. Fusion Energy Science Program] |
|||
==External links== |
|||
*[http://library.thinkquest.org/17940/texts/fusion_dt/fusion_dt.html] |
|||
*[http://www.iop.org/activity/policy/Publications/file_31695.pdf Fusion as an Energy Source]: A guide from the Institute of Physics |
|||
*[http://www.ofes.fusion.doe.gov/ U.S. Fusion Energy Science Program] |
|||
*[http://www.whatsnextnetwork.com/technology/index.php?s=fusion+energy&sentence=AND&submit=Search Latest Fusion Energy Research News] |
|||
*[http://www.fusion.org.uk/ EURATOM/UKAEA Fusion Association] |
|||
*[http://www.iter.org ITER] |
|||
*[http://fire.pppl.gov FIRE] |
|||
*[http://fusedweb.llnl.gov/faq.html FUSION FAQ] |
|||
*[http://www.efda.org European Fusion Development Agreement] |
|||
*[http://fusionpower.org/ Fusion Power Associates] A Washington, DC area lobbying organization; "a non-profit, tax-exempt research and educational foundation, providing timely information on the status of fusion development." Edits the ''Journal of Fusion Energy''. |
|||
*[http://fusedweb.pppl.gov/Glossary/glossary.html Plasma/Fusion Glossary] |
|||
*[http://www.ph.utexas.edu/%7Ephy315/Helimak.htm ''The Helimak Experiment'', at the Fusion Research Center at UT Austin] |
|||
*Investigations of the Formability, Weldability and Creep Resistance of Some Potential Low-activation Austenitic Stainless Steels for Fusion Reactor Applications (ISBN 0-85311-148-0): A.H. Bott, G.J. Butterworth, [[F. B. Pickering]] |
|||
*{{PDFlink|[http://www.fusion.org.uk/techdocs/euromat.pdf "Low Activation Material Candidates For Fusion Power Plants"; C.B.A. Forty and N.P. Taylor]|58.8 KB}} |
|||
*[http://news.bbc.co.uk/1/hi/sci/tech/5012638.stm International Thermonuclear Experimental Reactor (Iter) fusion reactor work gets go-ahead (BBC news May 2006)] |
|||
*[http://www.iterfan.org Unofficial ITER fan club] |
|||
*[http://europe.theoildrum.com/node/2164 Will Nuclear Fusion Fill the Gap Left by Peak Oil?] |
|||
*[http://www.ans.org/pubs/journals/fst/ Fusion Science and Technology] |
|||
*[http://video.google.com/videoplay?docid=1996321846673788606 Google Tech Talk] |
|||
*[http://www.generalfusion.com/ General Fusion--technology for a safe, economically viable modified MTF fusion reactor by 2010.] |
|||
*[http://www.intimal.edu.my/school/fas/UFLF/ Universal Plasma Focus Laboratory Facility] |
|||
*[http://www.fusionenergy.net.au A Central Site for Fusion Energy Links] |
|||
*[http://www.plasmafocus.net Institute for Plasma Focus Studies] |
|||
*{{Cite news|title= This Machine Might* Save the World |work=Popular Science |author=Josh Dean |date=December 23, 2008 |url=http://www.popsci.com/scitech/article/2008-12/machine-might-save-world }} |
|||
{{Fusion power}} |
{{Fusion power}} |
||
{{Nuclear Technology}} |
{{Nuclear Technology}} |
||
{{ |
{{emerging technologies|energy=yes}} |
||
{{DEFAULTSORT:Fusion Power}} |
|||
[[Category:Fusion power|*]] |
|||
[[Category:Emerging technology]] |
|||
[[Category:Alternative energy]] |
|||
[[Category:Hypothetical technology]] |
|||
[[Category:Fusion power| ]] |
|||
<!-- Interwiki --> |
|||
[[Category:Sustainable energy]] |
|||
[[ar:اندماج نووي]] |
|||
[[bn:তাপ-নিউক্লীয় বিক্রিয়ক]] |
|||
[[bg:Термоядрена енергия]] |
|||
[[ca:Energia de fusió]] |
|||
[[da:Fusionsenergi]] |
|||
[[de:Kernfusionsreaktor]] |
|||
[[es:Energía de fusión]] |
|||
[[fr:Énergie de fusion]] |
|||
[[id:Tenaga fusi]] |
|||
[[it:Energia da fusione]] |
|||
[[hu:Fúziós reaktor]] |
|||
[[ja:核融合エネルギー]] |
|||
[[pl:Kontrolowana synteza termojądrowa]] |
|||
[[ru:Управляемый термоядерный синтез]] |
|||
[[fi:Fuusioreaktori]] |
|||
[[sv:Fusionsenergi]] |
|||
[[th:ปฏิกิริยานิวเคลียร์ฟิวชัน]] |
|||
[[uk:Термоядерна енергія]] |
Latest revision as of 10:31, 23 June 2024

Fusion power is a proposed form of power generation that would generate electricity by using heat from nuclear fusion reactions. In a fusion process, two lighter atomic nuclei combine to form a heavier nucleus, while releasing energy. Devices designed to harness this energy are known as fusion reactors. Research into fusion reactors began in the 1940s, but as of 2024, no device has reached net power, although net positive reactions have been achieved.[1][2][3][4]
Fusion processes require fuel and a confined environment with sufficient temperature, pressure, and confinement time to create a plasma in which fusion can occur. The combination of these figures that results in a power-producing system is known as the Lawson criterion. In stars the most common fuel is hydrogen, and gravity provides extremely long confinement times that reach the conditions needed for fusion energy production. Proposed fusion reactors generally use heavy hydrogen isotopes such as deuterium and tritium (and especially a mixture of the two), which react more easily than protium (the most common hydrogen isotope) and produce a helium nucleus and an energized neutron[5], to allow them to reach the Lawson criterion requirements with less extreme conditions. Most designs aim to heat their fuel to around 100 million kelvins, which presents a major challenge in producing a successful design. Tritium is extremely rare on earth, having a half life of only ~12.3 years. Consequently, during the operation of envisioned fusion reactors, known as breeder reactors, helium cooled pebble beds (HCPBs) are subjected to neutron fluxes to generate tritium to complete the fuel cycle.[6]
As a source of power, nuclear fusion has a number of potential advantages compared to fission. These include reduced radioactivity in operation, little high-level nuclear waste, ample fuel supplies (assuming tritium breeding or some forms of aneutronic fuels), and increased safety. However, the necessary combination of temperature, pressure, and duration has proven to be difficult to produce in a practical and economical manner. A second issue that affects common reactions is managing neutrons that are released during the reaction, which over time degrade many common materials used within the reaction chamber.
Fusion researchers have investigated various confinement concepts. The early emphasis was on three main systems: z-pinch, stellarator, and magnetic mirror. The current leading designs are the tokamak and inertial confinement (ICF) by laser. Both designs are under research at very large scales, most notably the ITER tokamak in France and the National Ignition Facility (NIF) laser in the United States. Researchers are also studying other designs that may offer less expensive approaches. Among these alternatives, there is increasing interest in magnetized target fusion and inertial electrostatic confinement, and new variations of the stellarator.
Background[edit]


Mechanism[edit]
Fusion reactions occur when two or more atomic nuclei come close enough for long enough that the nuclear force pulling them together exceeds the electrostatic force pushing them apart, fusing them into heavier nuclei. For nuclei heavier than iron-56, the reaction is endothermic, requiring an input of energy.[7] The heavy nuclei bigger than iron have many more protons resulting in a greater repulsive force. For nuclei lighter than iron-56, the reaction is exothermic, releasing energy when they fuse. Since hydrogen has a single proton in its nucleus, it requires the least effort to attain fusion, and yields the most net energy output. Also since it has one electron, hydrogen is the easiest fuel to fully ionize.
The repulsive electrostatic interaction between nuclei operates across larger distances than the strong force, which has a range of roughly one femtometer—the diameter of a proton or neutron. The fuel atoms must be supplied enough kinetic energy to approach one another closely enough for the strong force to overcome the electrostatic repulsion in order to initiate fusion. The "Coulomb barrier" is the quantity of kinetic energy required to move the fuel atoms near enough. Atoms can be heated to extremely high temperatures or accelerated in a particle accelerator to produce this energy.
An atom loses its electrons once it is heated past its ionization energy. An ion is the name for the resultant bare nucleus. The result of this ionization is plasma, which is a heated cloud of ions and free electrons that were formerly bound to them. Plasmas are electrically conducting and magnetically controlled because the charges are separated. This is used by several fusion devices to confine the hot particles.
Cross section[edit]

A reaction's cross section, denoted σ, measures the probability that a fusion reaction will happen. This depends on the relative velocity of the two nuclei. Higher relative velocities generally increase the probability, but the probability begins to decrease again at very high energies.[8]
In a plasma, particle velocity can be characterized using a probability distribution. If the plasma is thermalized, the distribution looks like a Gaussian curve, or Maxwell–Boltzmann distribution. In this case, it is useful to use the average particle cross section over the velocity distribution. This is entered into the volumetric fusion rate:[9]
where:
- is the energy made by fusion, per time and volume
- n is the number density of species A or B, of the particles in the volume
- is the cross section of that reaction, average over all the velocities of the two species v
- is the energy released by that fusion reaction.
Lawson criterion[edit]
The Lawson criterion considers the energy balance between the energy produced in fusion reactions to the energy being lost to the environment. In order to generate usable energy, a system would have to produce more energy than it loses. Lawson assumed an energy balance, shown below.[9]
where:
- is the net power from fusion
- is the efficiency of capturing the output of the fusion
- is the rate of energy generated by the fusion reactions
- is the conduction losses as energetic mass leaves the plasma
- is the radiation losses as energy leaves as light.
The rate of fusion, and thus Pfusion, depends on the temperature and density of the plasma. The plasma loses energy through conduction and radiation.[9] Conduction occurs when ions, electrons, or neutrals impact other substances, typically a surface of the device, and transfer a portion of their kinetic energy to the other atoms. The rate of conduction is also based on the temperature and density. Radiation is energy that leaves the cloud as light. Radiation also increases with temperature as well as the mass of the ions. Fusion power systems must operate in a region where the rate of fusion is higher than the losses.
Triple product: density, temperature, time[edit]

The Lawson criterion argues that a machine holding a thermalized and quasi-neutral plasma has to generate enough energy to overcome its energy losses. The amount of energy released in a given volume is a function of the temperature, and thus the reaction rate on a per-particle basis, the density of particles within that volume, and finally the confinement time, the length of time that energy stays within the volume.[9][11] This is known as the "triple product": the plasma density, temperature, and confinement time.[12]
In magnetic confinement, the density is low, on the order of a "good vacuum". For instance, in the ITER device the fuel density is about 1.0 × 1019 m−3, which is about one-millionth atmospheric density.[13] This means that the temperature and/or confinement time must increase. Fusion-relevant temperatures have been achieved using a variety of heating methods that were developed in the early 1970s. In modern machines, as of 2019[update], the major remaining issue was the confinement time. Plasmas in strong magnetic fields are subject to a number of inherent instabilities, which must be suppressed to reach useful durations. One way to do this is to simply make the reactor volume larger, which reduces the rate of leakage due to classical diffusion. This is why ITER is so large.
In contrast, inertial confinement systems approach useful triple product values via higher density, and have short confinement intervals. In NIF, the initial frozen hydrogen fuel load has a density less than water that is increased to about 100 times the density of lead. In these conditions, the rate of fusion is so high that the fuel fuses in the microseconds it takes for the heat generated by the reactions to blow the fuel apart. Although NIF is also large, this is a function of its "driver" design, not inherent to the fusion process.
Energy capture[edit]
Multiple approaches have been proposed to capture the energy that fusion produces. The simplest is to heat a fluid. The commonly targeted D-T reaction releases much of its energy as fast-moving neutrons. Electrically neutral, the neutron is unaffected by the confinement scheme. In most designs, it is captured in a thick "blanket" of lithium surrounding the reactor core. When struck by a high-energy neutron, the blanket heats up. It is then actively cooled with a working fluid that drives a turbine to produce power.
Another design proposed to use the neutrons to breed fission fuel in a blanket of nuclear waste, a concept known as a fission-fusion hybrid. In these systems, the power output is enhanced by the fission events, and power is extracted using systems like those in conventional fission reactors.[14]
Designs that use other fuels, notably the proton-boron aneutronic fusion reaction, release much more of their energy in the form of charged particles. In these cases, power extraction systems based on the movement of these charges are possible. Direct energy conversion was developed at Lawrence Livermore National Laboratory (LLNL) in the 1980s as a method to maintain a voltage directly using fusion reaction products. This has demonstrated energy capture efficiency of 48 percent.[15]
Plasma behavior[edit]
Plasma is an ionized gas that conducts electricity.[16] In bulk, it is modeled using magnetohydrodynamics, which is a combination of the Navier–Stokes equations governing fluids and Maxwell's equations governing how magnetic and electric fields behave.[17] Fusion exploits several plasma properties, including:
- Self-organizing plasma conducts electric and magnetic fields. Its motions generate fields that can in turn contain it.[18]
- Diamagnetic plasma can generate its own internal magnetic field. This can reject an externally applied magnetic field, making it diamagnetic.[19]
- Magnetic mirrors can reflect plasma when it moves from a low to high density field.[20]:24
Methods[edit]

Magnetic confinement[edit]
- Tokamak: the most well-developed and well-funded approach. This method drives hot plasma around in a magnetically confined torus, with an internal current. When completed, ITER will become the world's largest tokamak. As of September 2018 an estimated 226 experimental tokamaks were either planned, decommissioned or operating (50) worldwide.[21]
- Spherical tokamak: also known as spherical torus. A variation on the tokamak with a spherical shape.
- Stellarator: Twisted rings of hot plasma. The stellarator attempts to create a natural twisted plasma path, using external magnets. Stellarators were developed by Lyman Spitzer in 1950 and evolved into four designs: Torsatron, Heliotron, Heliac and Helias. One example is Wendelstein 7-X, a German device. It is the world's largest stellarator.[22]
- Internal rings: Stellarators create a twisted plasma using external magnets, while tokamaks do so using a current induced in the plasma. Several classes of designs provide this twist using conductors inside the plasma. Early calculations showed that collisions between the plasma and the supports for the conductors would remove energy faster than fusion reactions could replace it. Modern variations, including the Levitated Dipole Experiment (LDX), use a solid superconducting torus that is magnetically levitated inside the reactor chamber.[23]
- Magnetic mirror: Developed by Richard F. Post and teams at Lawrence Livermore National Laboratory (LLNL) in the 1960s.[24] Magnetic mirrors reflect plasma back and forth in a line. Variations included the Tandem Mirror, magnetic bottle and the biconic cusp.[25] A series of mirror machines were built by the US government in the 1970s and 1980s, principally at LLNL.[26] However, calculations in the 1970s estimated it was unlikely these would ever be commercially useful.
- Bumpy torus: A number of magnetic mirrors are arranged end-to-end in a toroidal ring. Any fuel ions that leak out of one are confined in a neighboring mirror, permitting the plasma pressure to be raised arbitrarily high without loss. An experimental facility, the ELMO Bumpy Torus or EBT was built and tested at Oak Ridge National Laboratory (ORNL) in the 1970s.
- Field-reversed configuration: This device traps plasma in a self-organized quasi-stable structure; where the particle motion makes an internal magnetic field which then traps itself.[27]
- Spheromak: Similar to a field-reversed configuration, a semi-stable plasma structure made by using the plasmas' self-generated magnetic field. A spheromak has both toroidal and poloidal fields, while a field-reversed configuration has no toroidal field.[28]
- Dynomak is a spheromak that is formed and sustained using continuous magnetic flux injection.[29][30][31]
- Reversed field pinch: Here the plasma moves inside a ring. It has an internal magnetic field. Moving out from the center of this ring, the magnetic field reverses direction.
Inertial confinement[edit]

- Indirect drive: Lasers heat a structure known as a Hohlraum that becomes so hot it begins to radiate x-ray light. These x-rays heat a fuel pellet, causing it to collapse inward to compress the fuel. The largest system using this method is the National Ignition Facility, followed closely by Laser Mégajoule.[32]
- Direct drive: Lasers directly heat the fuel pellet. Notable direct drive experiments have been conducted at the Laboratory for Laser Energetics (LLE) and the GEKKO XII facilities. Good implosions require fuel pellets with close to a perfect shape in order to generate a symmetrical inward shock wave that produces the high-density plasma.[citation needed]
- Fast ignition: This method uses two laser blasts. The first blast compresses the fusion fuel, while the second ignites it. As of 2019[update] this technique had lost favor for energy production.[33]
- Magneto-inertial fusion or Magnetized Liner Inertial Fusion: This combines a laser pulse with a magnetic pinch. The pinch community refers to it as magnetized liner inertial fusion while the ICF community refers to it as magneto-inertial fusion.[34]
- Ion Beams: Ion beams replace laser beams to heat the fuel.[35] The main difference is that the beam has momentum due to mass, whereas lasers do not. As of 2019 it appears unlikely that ion beams can be sufficiently focused spatially and in time.
- Z-machine: Sends an electric current through thin tungsten wires, heating them sufficiently to generate x-rays. Like the indirect drive approach, these x-rays then compress a fuel capsule.
Magnetic or electric pinches[edit]
- Z-pinch: A current travels in the z-direction through the plasma. The current generates a magnetic field that compresses the plasma. Pinches were the first method for human-made controlled fusion.[36][37] The z-pinch has inherent instabilities that limit its compression and heating to values too low for practical fusion. The largest such machine, the UK's ZETA, was the last major experiment of the sort. The problems in z-pinch led to the tokamak design. The dense plasma focus is a possibly superior variation.
- Theta-pinch: A current circles around the outside of a plasma column, in the theta direction. This induces a magnetic field running down the center of the plasma, as opposed to around it. The early theta-pinch device Scylla was the first to conclusively demonstrate fusion, but later work demonstrated it had inherent limits that made it uninteresting for power production.
- Sheared Flow Stabilized Z-Pinch: Research at the University of Washington under Uri Shumlak investigated the use of sheared-flow stabilization to smooth out the instabilities of Z-pinch reactors. This involves accelerating neutral gas along the axis of the pinch. Experimental machines included the FuZE and Zap Flow Z-Pinch experimental reactors.[38] In 2017, British technology investor and entrepreneur Benj Conway, together with physicists Brian Nelson and Uri Shumlak, co-founded Zap Energy to attempt to commercialize the technology for power production.[39][40][41]
- Screw Pinch: This method combines a theta and z-pinch for improved stabilization.[42]
Inertial electrostatic confinement[edit]
- Fusor: An electric field heats ions to fusion conditions. The machine typically uses two spherical cages, a cathode inside the anode, inside a vacuum. These machines are not considered a viable approach to net power because of their high conduction and radiation losses.[43] They are simple enough to build that amateurs have fused atoms using them.[44]
- Polywell: Attempts to combine magnetic confinement with electrostatic fields, to avoid the conduction losses generated by the cage.[45]
Other[edit]
- Magnetized target fusion: Confines hot plasma using a magnetic field and squeezes it using inertia. Examples include LANL FRX-L machine,[46] General Fusion (piston compression with liquid metal liner), HyperJet Fusion (plasma jet compression with plasma liner).[47][48]
- Uncontrolled: Fusion has been initiated by man, using uncontrolled fission explosions to stimulate fusion. Early proposals for fusion power included using bombs to initiate reactions. See Project PACER.
- Beam fusion: A beam of high energy particles fired at another beam or target can initiate fusion. This was used in the 1970s and 1980s to study the cross sections of fusion reactions.[8] However beam systems cannot be used for power because keeping a beam coherent takes more energy than comes from fusion.
- Muon-catalyzed fusion: This approach replaces electrons in diatomic molecules of isotopes of hydrogen with muons—more massive particles with the same electric charge. Their greater mass compresses the nuclei enough such that the strong interaction can cause fusion.[49] As of 2007 producing muons required more energy than can be obtained from muon-catalyzed fusion.[50]
- Lattice confinement fusion: Lattice confinement fusion (LCF) is a type of nuclear fusion in which deuteron-saturated metals are exposed to gamma radiation or ion beams, such as in an IEC fusor, avoiding the confined high-temperature plasmas used in other methods of fusion.[51][52]
Common tools[edit]
Many approaches, equipment, and mechanisms are employed across multiple projects to address fusion heating, measurement, and power production.[53]
Neural networks[edit]
A deep reinforcement learning system has been used to control a tokamak-based reactor. The AI was able to manipulate the magnetic coils to manage the plasma. The system was able to continuously adjust to maintain appropriate behavior (more complex than step-based systems). In 2014, Google began working with California-based fusion company TAE Technologies to control the Joint European Torus (JET) to predict plasma behavior.[54] DeepMind has also developed a control scheme with TCV.[55]
Heating[edit]
- Electrostatic heating: an electric field can do work on charged ions or electrons, heating them.[56]
- Neutral beam injection: hydrogen is ionized and accelerated by an electric field to form a charged beam that is shone through a source of neutral hydrogen gas towards the plasma which itself is ionized and contained by a magnetic field. Some of the intermediate hydrogen gas is accelerated towards the plasma by collisions with the charged beam while remaining neutral: this neutral beam is thus unaffected by the magnetic field and so reaches the plasma. Once inside the plasma the neutral beam transmits energy to the plasma by collisions which ionize it and allow it to be contained by the magnetic field, thereby both heating and refueling the reactor in one operation. The remainder of the charged beam is diverted by magnetic fields onto cooled beam dumps.[57]
- Radio frequency heating: a radio wave causes the plasma to oscillate (i.e., microwave oven). This is also known as electron cyclotron resonance heating, using for example gyrotrons, or dielectric heating.[58]
- Magnetic reconnection: when plasma gets dense, its electromagnetic properties can change, which can lead to magnetic reconnection. Reconnection helps fusion because it instantly dumps energy into a plasma, heating it quickly. Up to 45% of the magnetic field energy can heat the ions.[59][60]
- Magnetic oscillations: varying electric currents can be supplied to magnetic coils that heat plasma confined within a magnetic wall.[61]
- Antiproton annihilation: antiprotons injected into a mass of fusion fuel can induce thermonuclear reactions. This possibility as a method of spacecraft propulsion, known as antimatter-catalyzed nuclear pulse propulsion, was investigated at Pennsylvania State University in connection with the proposed AIMStar project.[citation needed]
Measurement[edit]
The diagnostics of a fusion scientific reactor are extremely complex and varied.[62] The diagnostics required for a fusion power reactor will be various but less complicated than those of a scientific reactor as by the time of commercialization, many real-time feedback and control diagnostics will have been perfected. However, the operating environment of a commercial fusion reactor will be harsher for diagnostic systems than in a scientific reactor because continuous operations may involve higher plasma temperatures and higher levels of neutron irradiation. In many proposed approaches, commercialization will require the additional ability to measure and separate diverter gases, for example helium and impurities, and to monitor fuel breeding, for instance the state of a tritium breeding liquid lithium liner.[63] The following are some basic techniques.
- Flux loop: a loop of wire is inserted into the magnetic field. As the field passes through the loop, a current is made. The current measures the total magnetic flux through that loop. This has been used on the National Compact Stellarator Experiment,[64] the polywell,[65] and the LDX machines. A Langmuir probe, a metal object placed in a plasma, can be employed. A potential is applied to it, giving it a voltage against the surrounding plasma. The metal collects charged particles, drawing a current. As the voltage changes, the current changes. This makes an IV Curve. The IV-curve can be used to determine the local plasma density, potential and temperature.[66]
- Thomson scattering: "Light scatters" from plasma can be used to reconstruct plasma behavior, including density and temperature. It is common in Inertial confinement fusion,[67] Tokamaks,[68] and fusors. In ICF systems, firing a second beam into a gold foil adjacent to the target makes x-rays that traverse the plasma. In tokamaks, this can be done using mirrors and detectors to reflect light.
- Neutron detectors: Several types of neutron detectors can record the rate at which neutrons are produced.[69][70]
- X-ray detectors Visible, IR, UV, and X-rays are emitted anytime a particle changes velocity.[71] If the reason is deflection by a magnetic field, the radiation is cyclotron radiation at low speeds and synchrotron radiation at high speeds. If the reason is deflection by another particle, plasma radiates X-rays, known as Bremsstrahlung radiation.[72]
Power production[edit]
Neutron blankets absorb neutrons, which heats the blanket. Power can be extracted from the blanket in various ways:
- Steam turbines can be driven by heat transferred into a working fluid that turns into steam, driving electric generators.[73]
- Neutron blankets: These neutrons can regenerate spent fission fuel.[74] Tritium can be produced using a breeder blanket of liquid lithium or a helium cooled pebble bed made of lithium-bearing ceramic pebbles.[75]
- Direct conversion: The kinetic energy of a particle can be converted into voltage.[24] It was first suggested by Richard F. Post in conjunction with magnetic mirrors, in the late 1960s. It has been proposed for Field-Reversed Configurations as well as Dense Plasma Focus devices. The process converts a large fraction of the random energy of the fusion products into directed motion. The particles are then collected on electrodes at various large electrical potentials. This method has demonstrated an experimental efficiency of 48 percent.[76]
- Traveling-wave tubes pass charged helium atoms at several megavolts and just coming off the fusion reaction through a tube with a coil of wire around the outside. This passing charge at high voltage pulls electricity through the wire.
Confinement[edit]

Confinement refers to all the conditions necessary to keep a plasma dense and hot long enough to undergo fusion. General principles:
- Equilibrium: The forces acting on the plasma must be balanced. One exception is inertial confinement, where the fusion must occur faster than the dispersal time.
- Stability: The plasma must be constructed so that disturbances will not lead to the plasma dispersing.
- Transport or conduction: The loss of material must be sufficiently slow.[9] The plasma carries energy off with it, so rapid loss of material will disrupt fusion. Material can be lost by transport into different regions or conduction through a solid or liquid.
To produce self-sustaining fusion, part of the energy released by the reaction must be used to heat new reactants and maintain the conditions for fusion.
Magnetic confinement[edit]
Magnetic Mirror[edit]
Magnetic mirror effect. If a particle follows the field line and enters a region of higher field strength, the particles can be reflected. Several devices apply this effect. The most famous was the magnetic mirror machines, a series of devices built at LLNL from the 1960s to the 1980s.[77] Other examples include magnetic bottles and Biconic cusp.[78] Because the mirror machines were straight, they had some advantages over ring-shaped designs. The mirrors were easier to construct and maintain and direct conversion energy capture was easier to implement.[15] Poor confinement has led this approach to be abandoned, except in the polywell design.[79]
Magnetic loops[edit]
Magnetic loops bend the field lines back on themselves, either in circles or more commonly in nested toroidal surfaces. The most highly developed systems of this type are the tokamak, the stellarator, and the reversed field pinch. Compact toroids, especially the field-reversed configuration and the spheromak, attempt to combine the advantages of toroidal magnetic surfaces with those of a simply connected (non-toroidal) machine, resulting in a mechanically simpler and smaller confinement area.
Inertial confinement[edit]
Inertial confinement is the use of rapid implosion to heat and confine plasma. A shell surrounding the fuel is imploded using a direct laser blast (direct drive), a secondary x-ray blast (indirect drive), or heavy beams. The fuel must be compressed to about 30 times solid density with energetic beams. Direct drive can in principle be efficient, but insufficient uniformity has prevented success.[80]:19–20 Indirect drive uses beams to heat a shell, driving the shell to radiate x-rays, which then implode the pellet. The beams are commonly laser beams, but ion and electron beams have been investigated.[80]:182–193
Electrostatic confinement[edit]
Electrostatic confinement fusion devices use electrostatic fields. The best known is the fusor. This device has a cathode inside an anode wire cage. Positive ions fly towards the negative inner cage, and are heated by the electric field in the process. If they miss the inner cage they can collide and fuse. Ions typically hit the cathode, however, creating prohibitory high conduction losses. Fusion rates in fusors are low because of competing physical effects, such as energy loss in the form of light radiation.[81] Designs have been proposed to avoid the problems associated with the cage, by generating the field using a non-neutral cloud. These include a plasma oscillating device,[82] a magnetically shielded-grid,[83] a penning trap, the polywell,[84] and the F1 cathode driver concept.[85]
Fuels[edit]
The fuels considered for fusion power have all been light elements like the isotopes of hydrogen—protium, deuterium, and tritium.[8] The deuterium and helium-3 reaction requires helium-3, an isotope of helium so scarce on Earth that it would have to be mined extraterrestrially or produced by other nuclear reactions. Ultimately, researchers hope to adopt the protium–boron-11 reaction, because it does not directly produce neutrons, although side reactions can.[86]
Deuterium, tritium[edit]
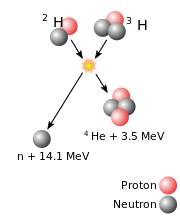
The easiest nuclear reaction, at the lowest energy, is D+T:
This reaction is common in research, industrial and military applications, usually as a neutron source. Deuterium is a naturally occurring isotope of hydrogen and is commonly available. The large mass ratio of the hydrogen isotopes makes their separation easy compared to the uranium enrichment process. Tritium is a natural isotope of hydrogen, but because it has a short half-life of 12.32 years, it is hard to find, store, produce, and is expensive. Consequently, the deuterium-tritium fuel cycle requires the breeding of tritium from lithium using one of the following reactions:
- 1
0n
+ 6
3Li
→ 3
1T
+ 4
2He - 1
0n
+ 7
3Li
→ 3
1T
+ 4
2He
+ 1
0n
The reactant neutron is supplied by the D-T fusion reaction shown above, and the one that has the greatest energy yield. The reaction with 6Li is exothermic, providing a small energy gain for the reactor. The reaction with 7Li is endothermic, but does not consume the neutron. Neutron multiplication reactions are required to replace the neutrons lost to absorption by other elements. Leading candidate neutron multiplication materials are beryllium and lead, but the 7Li reaction helps to keep the neutron population high. Natural lithium is mainly 7Li, which has a low tritium production cross section compared to 6Li so most reactor designs use breeding blankets with enriched 6Li.
Drawbacks commonly attributed to D-T fusion power include:
- The supply of neutrons results in neutron activation of the reactor materials.[87]:242
- 80% of the resultant energy is carried off by neutrons, which limits the use of direct energy conversion.[88]
- It requires the radioisotope tritium. Tritium may leak from reactors. Some estimates suggest that this would represent a substantial environmental radioactivity release.[89]
The neutron flux expected in a commercial D-T fusion reactor is about 100 times that of fission power reactors, posing problems for material design. After a series of D-T tests at JET, the vacuum vessel was sufficiently radioactive that it required remote handling for the year following the tests.[90]
In a production setting, the neutrons would react with lithium in the breeding blanket composed of lithium ceramic pebbles or liquid lithium, yielding tritium. The energy of the neutrons ends up in the lithium, which would then be transferred to drive electrical production. The lithium blanket protects the outer portions of the reactor from the neutron flux. Newer designs, the advanced tokamak in particular, use lithium inside the reactor core as a design element. The plasma interacts directly with the lithium, preventing a problem known as "recycling". The advantage of this design was demonstrated in the Lithium Tokamak Experiment.
Deuterium[edit]

Fusing two deuterium nuclei is the second easiest fusion reaction. The reaction has two branches that occur with nearly equal probability:
D + D → T + 1H D + D → 3He + n
This reaction is also common in research. The optimum energy to initiate this reaction is 15 keV, only slightly higher than that for the D-T reaction. The first branch produces tritium, so that a D-D reactor is not tritium-free, even though it does not require an input of tritium or lithium. Unless the tritons are quickly removed, most of the tritium produced is burned in the reactor, which reduces the handling of tritium, with the disadvantage of producing more, and higher-energy, neutrons. The neutron from the second branch of the D-D reaction has an energy of only 2.45 MeV (0.393 pJ), while the neutron from the D-T reaction has an energy of 14.1 MeV (2.26 pJ), resulting in greater isotope production and material damage. When the tritons are removed quickly while allowing the 3He to react, the fuel cycle is called "tritium suppressed fusion".[91] The removed tritium decays to 3He with a 12.5 year half life. By recycling the 3He decay into the reactor, the fusion reactor does not require materials resistant to fast neutrons.
Assuming complete tritium burn-up, the reduction in the fraction of fusion energy carried by neutrons would be only about 18%, so that the primary advantage of the D-D fuel cycle is that tritium breeding is not required. Other advantages are independence from lithium resources and a somewhat softer neutron spectrum. The disadvantage of D-D compared to D-T is that the energy confinement time (at a given pressure) must be 30 times longer and the power produced (at a given pressure and volume) is 68 times less.[citation needed]
Assuming complete removal of tritium and 3He recycling, only 6% of the fusion energy is carried by neutrons. The tritium-suppressed D-D fusion requires an energy confinement that is 10 times longer compared to D-T and double the plasma temperature.[92]
Deuterium, helium-3[edit]
A second-generation approach to controlled fusion power involves combining helium-3 (3He) and deuterium (2H):
D + 3He → 4He + 1H
This reaction produces 4He and a high-energy proton. As with the p-11B aneutronic fusion fuel cycle, most of the reaction energy is released as charged particles, reducing activation of the reactor housing and potentially allowing more efficient energy harvesting (via any of several pathways).[93] In practice, D-D side reactions produce a significant number of neutrons, leaving p-11B as the preferred cycle for aneutronic fusion.[93]
Proton, boron-11[edit]
Both material science problems and non-proliferation concerns are greatly diminished by aneutronic fusion. Theoretically, the most reactive aneutronic fuel is 3He. However, obtaining reasonable quantities of 3He implies large scale extraterrestrial mining on the Moon or in the atmosphere of Uranus or Saturn. Therefore, the most promising candidate fuel for such fusion is fusing the readily available protium (i.e. a proton) and boron. Their fusion releases no neutrons, but produces energetic charged alpha (helium) particles whose energy can directly be converted to electrical power:
- p + 11B → 3 4He
Side reactions are likely to yield neutrons that carry only about 0.1% of the power,[94]:177–182 which means that neutron scattering is not used for energy transfer and material activation is reduced several thousand-fold. The optimum temperature for this reaction of 123 keV[95] is nearly ten times higher than that for pure hydrogen reactions, and energy confinement must be 500 times better than that required for the D-T reaction. In addition, the power density is 2500 times lower than for D-T, although per unit mass of fuel, this is still considerably higher compared to fission reactors.
Because the confinement properties of the tokamak and laser pellet fusion are marginal, most proposals for aneutronic fusion are based on radically different confinement concepts, such as the Polywell and the Dense Plasma Focus. In 2013, a research team led by Christine Labaune at École Polytechnique, reported a new fusion rate record for proton-boron fusion, with an estimated 80 million fusion reactions during a 1.5 nanosecond laser fire, 100 times greater than reported in previous experiments.[96][97]
Material selection[edit]
Structural material stability is a critical issue.[98][99] Materials that can survive the high temperatures and neutron bombardment experienced in a fusion reactor are considered key to success.[100][98] The principal issues are the conditions generated by the plasma, neutron degradation of wall surfaces, and the related issue of plasma-wall surface conditions.[101][102] Reducing hydrogen permeability is seen as crucial to hydrogen recycling[103] and control of the tritium inventory.[104] Materials with the lowest bulk hydrogen solubility and diffusivity provide the optimal candidates for stable barriers. A few pure metals, including tungsten and beryllium,[105] and compounds such as carbides, dense oxides, and nitrides have been investigated. Research has highlighted that coating techniques for preparing well-adhered and perfect barriers are of equivalent importance. The most attractive techniques are those in which an ad-layer is formed by oxidation alone. Alternative methods utilize specific gas environments with strong magnetic and electric fields. Assessment of barrier performance represents an additional challenge. Classical coated membranes gas permeation continues to be the most reliable method to determine hydrogen permeation barrier (HPB) efficiency.[104] In 2021, in response to increasing numbers of designs for fusion power reactors for 2040, the United Kingdom Atomic Energy Authority published the UK Fusion Materials Roadmap 2021–2040, focusing on five priority areas, with a focus on tokamak family reactors:
- Novel materials to minimize the amount of activation in the structure of the fusion power plant;
- Compounds that can be used within the power plant to optimise breeding of tritium fuel to sustain the fusion process;
- Magnets and insulators that are resistant to irradiation from fusion reactions—especially under cryogenic conditions;
- Structural materials able to retain their strength under neutron bombardment at high operating temperatures (over 550 degrees C);
- Engineering assurance for fusion materials—providing irradiated sample data and modelled predictions such that plant designers, operators and regulators have confidence that materials are suitable for use in future commercial power stations.
Superconducting materials[edit]

In a plasma that is embedded in a magnetic field (known as a magnetized plasma) the fusion rate scales as the magnetic field strength to the 4th power. For this reason, many fusion companies that rely on magnetic fields to control their plasma are trying to develop high temperature superconducting devices. In 2021, SuperOx, a Russian and Japanese company, developed a new manufacturing process for making superconducting YBCO wire for fusion reactors. This new wire was shown to conduct between 700 and 2000 Amps per square millimeter. The company was able to produce 186 miles of wire in nine months.[106]
Containment considerations[edit]
Even on smaller production scales, the containment apparatus is blasted with matter and energy. Designs for plasma containment must consider:
- A heating and cooling cycle, up to a 10 MW/m2 thermal load.
- Neutron radiation, which over time leads to neutron activation and embrittlement.
- High energy ions leaving at tens to hundreds of electronvolts.
- Alpha particles leaving at millions of electronvolts.
- Electrons leaving at high energy.
- Light radiation (IR, visible, UV, X-ray).
Depending on the approach, these effects may be higher or lower than fission reactors.[107] One estimate put the radiation at 100 times that of a typical pressurized water reactor.[citation needed] Depending on the approach, other considerations such as electrical conductivity, magnetic permeability, and mechanical strength matter. Materials must also not end up as long-lived radioactive waste.[98]
Plasma-wall surface conditions[edit]
For long term use, each atom in the wall is expected to be hit by a neutron and displaced about 100 times before the material is replaced. High-energy neutrons produce hydrogen and helium via nuclear reactions that tend to form bubbles at grain boundaries and result in swelling, blistering or embrittlement.[107]
Selection of materials[edit]
Low-Z materials, such as graphite or beryllium are generally preferred to high-Z materials, usually tungsten with molybdenum as a second choice.[104] Liquid metals (lithium, gallium, tin) have been proposed, e.g., by injection of 1–5 mm thick streams flowing at 10 m/s on solid substrates.[citation needed]
Graphite features a gross erosion rate due to physical and chemical sputtering amounting to many meters per year, requiring redeposition of the sputtered material. The redeposition site generally does not exactly match the sputter site, allowing net erosion that may be prohibitive. An even larger problem is that tritium is redeposited with the redeposited graphite. The tritium inventory in the wall and dust could build up to many kilograms, representing a waste of resources and a radiological hazard in case of an accident. Graphite found favor as material for short-lived experiments, but appears unlikely to become the primary plasma-facing material (PFM) in a commercial reactor.[98][108]
Tungsten's sputtering rate is orders of magnitude smaller than carbon's, and tritium is much less incorporated into redeposited tungsten. However, tungsten plasma impurities are much more damaging than carbon impurities, and self-sputtering can be high, requiring the plasma in contact with the tungsten not be too hot (a few tens of eV rather than hundreds of eV). Tungsten also has issues around eddy currents and melting in off-normal events, as well as some radiological issues.[98]
Safety and the environment[edit]
Accident potential[edit]
Accident potential and effect on the environment are critical to social acceptance of nuclear fusion, also known as a social license.[109] Fusion reactors are not subject to catastrophic meltdown.[110] It requires precise and controlled temperature, pressure and magnetic field parameters to produce net energy, and any damage or loss of required control would rapidly quench the reaction.[111] Fusion reactors operate with seconds or even microseconds worth of fuel at any moment. Without active refueling, the reactions immediately quench.[110]
The same constraints prevent runaway reactions. Although the plasma is expected to have a volume of 1,000 m3 (35,000 cu ft) or more, the plasma typically contains only a few grams of fuel.[110] By comparison, a fission reactor is typically loaded with enough fuel for months or years, and no additional fuel is necessary to continue the reaction. This large fuel supply is what offers the possibility of a meltdown.[112]
In magnetic containment, strong fields develop in coils that are mechanically held in place by the reactor structure. Failure of this structure could release this tension and allow the magnet to "explode" outward. The severity of this event would be similar to other industrial accidents or an MRI machine quench/explosion, and could be effectively contained within a containment building similar to those used in fission reactors.
In laser-driven inertial containment the larger size of the reaction chamber reduces the stress on materials. Although failure of the reaction chamber is possible, stopping fuel delivery prevents catastrophic failure.[113]
Most reactor designs rely on liquid hydrogen as a coolant and to convert stray neutrons into tritium, which is fed back into the reactor as fuel. Hydrogen is flammable, and it is possible that hydrogen stored on-site could ignite. In this case, the tritium fraction of the hydrogen would enter the atmosphere, posing a radiation risk. Calculations suggest that about 1 kilogram (2.2 lb) of tritium and other radioactive gases in a typical power station would be present. The amount is small enough that it would dilute to legally acceptable limits by the time they reached the station's perimeter fence.[114]
The likelihood of small industrial accidents, including the local release of radioactivity and injury to staff, are estimated to be minor compared to fission. They would include accidental releases of lithium or tritium or mishandling of radioactive reactor components.[113]
Magnet quench[edit]
A magnet quench is an abnormal termination of magnet operation that occurs when part of the superconducting coil exits the superconducting state (becomes normal). This can occur because the field inside the magnet is too large, the rate of change of field is too large (causing eddy currents and resultant heating in the copper support matrix), or a combination of the two.
More rarely a magnet defect can cause a quench. When this happens, that particular spot is subject to rapid Joule heating from the current, which raises the temperature of the surrounding regions. This pushes those regions into the normal state as well, which leads to more heating in a chain reaction. The entire magnet rapidly becomes normal over several seconds, depending on the size of the superconducting coil. This is accompanied by a loud bang as the energy in the magnetic field is converted to heat, and the cryogenic fluid boils away. The abrupt decrease of current can result in kilovolt inductive voltage spikes and arcing. Permanent damage to the magnet is rare, but components can be damaged by localized heating, high voltages, or large mechanical forces.
In practice, magnets usually have safety devices to stop or limit the current when a quench is detected. If a large magnet undergoes a quench, the inert vapor formed by the evaporating cryogenic fluid can present a significant asphyxiation hazard to operators by displacing breathable air.
A large section of the superconducting magnets in CERN's Large Hadron Collider unexpectedly quenched during start-up operations in 2008, destroying multiple magnets.[115] In order to prevent a recurrence, the LHC's superconducting magnets are equipped with fast-ramping heaters that are activated when a quench event is detected. The dipole bending magnets are connected in series. Each power circuit includes 154 individual magnets, and should a quench event occur, the entire combined stored energy of these magnets must be dumped at once. This energy is transferred into massive blocks of metal that heat up to several hundred degrees Celsius—because of resistive heating—in seconds. A magnet quench is a "fairly routine event" during the operation of a particle accelerator.[116]
Effluents[edit]
The natural product of the fusion reaction is a small amount of helium, which is harmless to life. Hazardous tritium is difficult to retain completely.
Although tritium is volatile and biologically active, the health risk posed by a release is much lower than that of most radioactive contaminants, because of tritium's short half-life (12.32 years) and very low decay energy (~14.95 keV), and because it does not bioaccumulate (it cycles out of the body as water, with a biological half-life of 7 to 14 days).[117] ITER incorporates total containment facilities for tritium.[118]
Radioactive waste[edit]
Fusion reactors create far less radioactive material than fission reactors. Further, the material it creates is less damaging biologically, and the radioactivity dissipates within a time period that is well within existing engineering capabilities for safe long-term waste storage.[119] In specific terms, except in the case of aneutronic fusion,[120][121] the neutron flux turns the structural materials radioactive. The amount of radioactive material at shut-down may be comparable to that of a fission reactor, with important differences. The half-lives of fusion and neutron activation radioisotopes tend to be less than those from fission, so that the hazard decreases more rapidly. Whereas fission reactors produce waste that remains radioactive for thousands of years, the radioactive material in a fusion reactor (other than tritium) would be the reactor core itself and most of this would be radioactive for about 50 years, with other low-level waste being radioactive for another 100 years or so thereafter.[122] The fusion waste's short half-life eliminates the challenge of long-term storage. By 500 years, the material would have the same radiotoxicity as coal ash.[114] Nonetheless, classification as intermediate level waste rather than low-level waste may complicate safety discussions.[123][119]
The choice of materials is less constrained than in conventional fission, where many materials are required for their specific neutron cross-sections. Fusion reactors can be designed using "low activation", materials that do not easily become radioactive. Vanadium, for example, becomes much less radioactive than stainless steel.[124] Carbon fiber materials are also low-activation, are strong and light, and are promising for laser-inertial reactors where a magnetic field is not required.[125]
Nuclear proliferation[edit]
In some scenarios, fusion power technology could be adapted to produce materials for military purposes. A huge amount of tritium could be produced by a fusion power station; tritium is used in the trigger of hydrogen bombs and in modern boosted fission weapons, but it can be produced in other ways. The energetic neutrons from a fusion reactor could be used to breed weapons-grade plutonium or uranium for an atomic bomb (for example by transmutation of 238
U to 239
Pu, or 232
Th to 233
U).
A study conducted in 2011 assessed three scenarios:[126]
- Small-scale fusion station: As a result of much higher power consumption, heat dissipation and a more recognizable design compared to enrichment gas centrifuges, this choice would be much easier to detect and therefore implausible.[126]
- Commercial facility: The production potential is significant. But no fertile or fissile substances necessary for the production of weapon-usable materials needs to be present at a civil fusion system at all. If not shielded, detection of these materials can be done by their characteristic gamma radiation. The underlying redesign could be detected by regular design information verification. In the (technically more feasible) case of solid breeder blanket modules, it would be necessary for incoming components to be inspected for the presence of fertile material,[126] otherwise plutonium for several weapons could be produced each year.[127]
- Prioritizing weapon-grade material regardless of secrecy: The fastest way to produce weapon-usable material was seen in modifying a civil fusion power station. No weapons-compatible material is required during civil use. Even without the need for covert action, such a modification would take about two months to start production and at least an additional week to generate a significant amount. This was considered to be enough time to detect a military use and to react with diplomatic or military means. To stop the production, a military destruction of parts of the facility while leaving out the reactor would be sufficient.[126]
Another study concluded "...large fusion reactors—even if not designed for fissile material breeding—could easily produce several hundred kg Pu per year with high weapon quality and very low source material requirements." It was emphasized that the implementation of features for intrinsic proliferation resistance might only be possible at an early phase of research and development.[127] The theoretical and computational tools needed for hydrogen bomb design are closely related to those needed for inertial confinement fusion, but have very little in common with magnetic confinement fusion.
Fuel reserves[edit]
Fusion power commonly proposes the use of deuterium as fuel and many current designs also use lithium. Assuming a fusion energy output equal to the 1995 global power output of about 100 EJ/yr (= 1 × 1020 J/yr) and that this does not increase in the future, which is unlikely, then known current lithium reserves would last 3000 years. Lithium from sea water would last 60 million years, however, and a more complicated fusion process using only deuterium would have fuel for 150 billion years.[128] To put this in context, 150 billion years is close to 30 times the remaining lifespan of the Sun,[129] and more than 10 times the estimated age of the universe.
Economics[edit]
The EU spent almost €10 billion through the 1990s.[130] ITER represents an investment of over twenty billion dollars, and possibly tens of billions more, including in kind contributions.[131][132] Under the European Union's Sixth Framework Programme, nuclear fusion research received €750 million (in addition to ITER funding), compared with €810 million for sustainable energy research,[133] putting research into fusion power well ahead of that of any single rival technology. The United States Department of Energy has allocated $US367M–$US671M every year since 2010, peaking in 2020,[134] with plans to reduce investment to $US425M in its FY2021 Budget Request.[135] About a quarter of this budget is directed to support ITER.
The size of the investments and time lines meant that fusion research was traditionally almost exclusively publicly funded. However, starting in the 2010s, the promise of commercializing a paradigm-changing low-carbon energy source began to attract a raft of companies and investors.[136] Over two dozen start-up companies attracted over one billion dollars from roughly 2000 to 2020, mainly from 2015, and a further three billion in funding and milestone related commitments in 2021,[137][138] with investors including Jeff Bezos, Peter Thiel and Bill Gates, as well as institutional investors including Legal & General, and energy companies including Equinor, Eni, Chevron,[139] and the Chinese ENN Group.[140][141][142] In 2021, Commonwealth Fusion Systems (CFS) obtained $1.8 billion in scale-up funding, and Helion Energy obtained a half-billion dollars with an additional $1.7 billion contingent on meeting milestones.[143]
Scenarios developed in the 2000s and early 2010s discussed the effects of the commercialization of fusion power on the future of human civilization.[144] Using nuclear fission as a guide, these saw ITER and later DEMO as bringing online the first commercial reactors around 2050 and a rapid expansion after mid-century.[144] Some scenarios emphasized "fusion nuclear science facilities" as a step beyond ITER.[145][146] However, the economic obstacles to tokamak-based fusion power remain immense, requiring investment to fund prototype tokamak reactors[147] and development of new supply chains,[148] a problem which will affect any kind of fusion reactor.[149] Tokamak designs appear to be labour-intensive,[150] while the commercialization risk of alternatives like inertial fusion energy is high due to the lack of government resources.[151]
Scenarios since 2010 note computing and material science advances enabling multi-phase national or cost-sharing "Fusion Pilot Plants" (FPPs) along various technology pathways,[152][146][153][154][155][156] such as the UK Spherical Tokamak for Energy Production, within the 2030–2040 time frame.[157][158][159] Notably, in June 2021, General Fusion announced it would accept the UK government's offer to host the world's first substantial public-private partnership fusion demonstration plant, at Culham Centre for Fusion Energy.[160] The plant will be constructed from 2022 to 2025 and is intended to lead the way for commercial pilot plants in the late 2025s. The plant will be 70% of full scale and is expected to attain a stable plasma of 150 million degrees.[161] In the United States, cost-sharing public-private partnership FPPs appear likely,[162] and in 2022 the DOE announced a new Milestone-Based Fusion Development Program as the centerpiece of its Bold Decadal Vision for Commercial Fusion Energy,[163] which envisages private sector-led teams delivering FPP pre-conceptual designs, defining technology roadmaps, and pursuing the R&D necessary to resolve critical-path scientific and technical issues towards an FPP design.[164] Compact reactor technology based on such demonstration plants may enable commercialization via a fleet approach from the 2030s[165] if early markets can be located.[159]
The widespread adoption of non-nuclear renewable energy has transformed the energy landscape. Such renewables are projected to supply 74% of global energy by 2050.[166] The steady fall of renewable energy prices challenges the economic competitiveness of fusion power.[167]

Some economists suggest fusion power is unlikely to match other renewable energy costs.[167] Fusion plants are expected to face large start up and capital costs. Moreover, operation and maintenance are likely to be costly.[167] While the costs of the China Fusion Engineering Test Reactor are not well known, an EU DEMO fusion concept was projected to feature a levelized cost of energy (LCOE) of $121/MWh.[169]
Fuel costs are low, but economists suggest that the energy cost for a one-gigawatt plant would increase by $16.5 per MWh for every $1 billion increase in the capital investment in construction. There is also the risk that easily obtained lithium will be used up making batteries. Obtaining it from seawater would be very costly and might require more energy than the energy that would be generated.[167]
In contrast, renewable levelized cost of energy estimates are substantially lower. For instance, the 2019 levelized cost of energy of solar energy was estimated to be $40-$46/MWh, on shore wind was estimated at $29-$56/MWh, and offshore wind was approximately $92/MWh.[170]
However, fusion power may still have a role filling energy gaps left by renewables,[159][167] depending on how administration priorities for energy and environmental justice influence the market.[143] In the 2020s, socioeconomic studies of fusion that began to consider these factors emerged,[171] and in 2022 EUROFusion launched its Socio-Economic Studies and Prospective Research and Development strands to investigate how such factors might affect commercialization pathways and timetables.[172] Similarly, in April 2023 Japan announced a national strategy to industrialise fusion.[173] Thus, fusion power may work in tandem with other renewable energy sources rather than becoming the primary energy source.[167] In some applications, fusion power could provide the base load, especially if including integrated thermal storage and cogeneration and considering the potential for retrofitting coal plants.[159][167]
Regulation[edit]
As fusion pilot plants move within reach, legal and regulatory issues must be addressed.[174] In September 2020, the United States National Academy of Sciences consulted with private fusion companies to consider a national pilot plant. The following month, the United States Department of Energy, the Nuclear Regulatory Commission (NRC) and the Fusion Industry Association co-hosted a public forum to begin the process.[139] In November 2020, the International Atomic Energy Agency (IAEA) began working with various nations to create safety standards[175] such as dose regulations and radioactive waste handling.[175] In January and March 2021, NRC hosted two public meetings on regulatory frameworks.[176][177] A public-private cost-sharing approach was endorsed in the 27 December H.R.133 Consolidated Appropriations Act, 2021, which authorized $325 million over five years for a partnership program to build fusion demonstration facilities, with a 100% match from private industry.[178]
Subsequently, the UK Regulatory Horizons Council published a report calling for a fusion regulatory framework by early 2022[179] in order to position the UK as a global leader in commercializing fusion power.[180] This call was met by the UK government publishing in October 2021 both its Fusion Green Paper and its Fusion Strategy, to regulate and commercialize fusion, respectively.[181][182][183] Then, in April 2023, in a decision likely to influence other nuclear regulators, the NRC announced in a unanimous vote that fusion energy would be regulated not as fission but under the same regulatory regime as particle accelerators.[184]
Then, in October 2023 the UK government, in enacting the Energy Act 2023, made the UK the first country to legislate for fusion separately from fission, to support planning and investment, including the UK's planned prototype fusion power plant for 2040; STEP[185] the UK is working with Canada and Japan in this regard.[186] Meanwhile, in February 2024 the US House of Representatives passed the Atomic Energy Advancement Act, which includes the Fusion Energy Act, which establishes a regulatory framework for fusion energy systems.[187]
Geopolitics[edit]
Given the potential of fusion to transform the world's energy industry and mitigate climate change,[188][189] fusion science has traditionally been seen as an integral part of peace-building science diplomacy.[190][118] However, technological developments[191] and private sector involvement has raised concerns over intellectual property, regulatory administration, global leadership;[188] equity, and potential weaponization.[142][192] These challenge ITER's peace-building role and led to calls for a global commission.[192][193] Fusion power significantly contributing to climate change by 2050 seems unlikely without substantial breakthroughs and a space race mentality emerging,[153][194] but a contribution by 2100 appears possible, with the extent depending on the type and particularly cost of technology pathways.[195][196]
Developments from late 2020 onwards have led to talk of a "new space race" with multiple entrants, pitting the US against China[48] and the UK's STEP FPP.[197][198] On 24 September 2020, the United States House of Representatives approved a research and commercialization program. The Fusion Energy Research section incorporated a milestone-based, cost-sharing, public-private partnership program modeled on NASA's COTS program, which launched the commercial space industry.[139] In February 2021, the National Academies published Bringing Fusion to the U.S. Grid, recommending a market-driven, cost-sharing plant for 2035–2040,[199][200][201] and the launch of the Congressional Bipartisan Fusion Caucus followed.[202]
In December 2020, an independent expert panel reviewed EUROfusion's design and R&D work on DEMO, and EUROfusion confirmed it was proceeding with its Roadmap to Fusion Energy, beginning the conceptual design of DEMO in partnership with the European fusion community, suggesting an EU-backed machine had entered the race.[203]
In November 2023 the UK and the US announced a bilateral partnership to accelerate fusion energy. Then, in December 2023 at COP28 the US announced a US global strategy to commercialize fusion energy.[204] Then, in April 2024, Japan and the US announced a similar partnership,[205] and in May of the same year, the G7 announced a G7 Working Group on Fusion Energy to promote international collaborations to accelerate the development of commercial energy and promote R&D between countries, as well as rationalize fusion regulation.[206]
Specifically to resolve the tritium supply problem, in February 2024, the UK (UKAEA) and Canada (Canadian Nuclear Laboratories) announced an agreement by which Canada could refurbish its Candu deuterium-uranium tritium-generating heavywater nuclear plants and even build new ones, guaranteeing a supply of tritium into the 2070s, while the UKAEA would test breeder materials and simulate how tritium could be captured, purified, and injected back into the fusion reaction.[207]
Advantages[edit]
Fusion power promises to provide more energy for a given weight of fuel than any fuel-consuming energy source currently in use.[208] The fuel (primarily deuterium) exists abundantly in the ocean: about 1 in 6500 hydrogen atoms in seawater is deuterium.[209] Although this is only about 0.015%, seawater is plentiful and easy to access, implying that fusion could supply the world's energy needs for millions of years.[210][211]
First generation fusion plants are expected to use the deuterium-tritium fuel cycle. This will require the use of lithium for breeding of the tritium. It is not known for how long global lithium supplies will suffice to supply this need as well as those of the battery and metallurgical industries. It is expected that second generation plants will move on to the more formidable deuterium-deuterium reaction. The deuterium-helium-3 reaction is also of interest, but the light helium isotope is practically non-existent on Earth. It is thought to exist in useful quantities in the lunar regolith, and is abundant in the atmospheres of the gas giant planets.
Fusion power could be used for so-called "deep space" propulsion within the solar system[212][213] and for interstellar space exploration where solar energy is not available, including via antimatter-fusion hybrid drives.[214][215]
Disadvantages[edit]
Fusion power has a number of disadvantages. Because 80 percent of the energy in any reactor fueled by deuterium and tritium appears in the form of neutron streams, such reactors share many of the drawbacks of fission reactors. This includes the production of large quantities of radioactive waste and serious radiation damage to reactor components. Additionally, naturally occurring tritium is extremely rare. While the hope is that fusion reactors can breed their own tritium, tritium self-sufficiency is extremely challenging, not least because tritium is difficult to contain (tritium has leaked from 48 of 65 nuclear sites in the US[216]). In any case the reserve and start-up tritium inventory requirements are likely to be unacceptably large.[217]
If reactors can be made to operate using only deuterium fuel, then the tritium replenishment issue is eliminated and neutron radiation damage may be reduced. However, the probabilities of deuterium-deuterium reactions are about 20 times lower than for deuterium-tritium. Additionally, the temperature needed is about 3 times higher than for deuterium-tritium (see cross section). The higher temperatures and lower reaction rates thus significantly complicate the engineering challenges. In any case, other drawbacks remain, for instance reactors requiring only deuterium fueling will have greatly enhanced nuclear weapons proliferation potential.
History[edit]
Early experiments[edit]


The first machine to achieve controlled thermonuclear fusion was a pinch machine at Los Alamos National Laboratory called Scylla I at the start of 1958. The team that achieved it was led by a British scientist named James Tuck and included a young Marshall Rosenbluth. Tuck had been involved in the Manhattan project, but had switched to working on fusion in the early 1950s. He applied for funding for the project as part of a White House sponsored contest to develop a fusion reactor along with Lyman Spitzer. Spitzer went on to develop the Stellarator concept at Princeton. The previous year, 1957, the British had claimed that they had achieved thermonuclear fusion reactions on the Zeta pinch machine. However, it turned out that the neutrons they had detected were from beam-target interactions, not fusion, and they withdrew the claim.
Scylla I was a classified machine at the time, so the fact that humankind had demonstrated controlled nuclear fusion in bulk was hidden from the public. A traditional Z-pinches passes a current down the center of a plasma, which makes a magnetic force around the outside which squeezes the plasma to fusion conditions. Scylla I was a twist on this basic idea; it was a θ-pinch, which passes a current around the outside creating a magnetic force in the center [37] Scylla I was a θ-pinch machine, with a cylinder full of deuterium.[36][37] After the success of Scylla-I, Los Alamos went on to build multiple pinch machines over the next few years. But the problem with pinches were that they were unstable because of a whole host of instabilities.
Lots of fusion approaches were pursued in parallel during this period and Princeton emerged as a hub for Stellarator research. It was pioneered by Lyman Spitzer. While fusion did not immediately transpire, the effort led to the creation of the Princeton Plasma Physics Laboratory.[218][219]
First tokamak[edit]
The concept of the tokamak originated in 1950–1951 from I.E. Tamm and A.D. Sakharov in the Soviet Union. The tokamak essentially combined a low-power pinch device with a low-power stellarator.[190] A.D. Sakharov's group constructed the first tokamaks, achieving the first quasistationary fusion reaction.[220]:90
Over time the "advanced tokamak" concept emerged, which included non-circular plasma, internal diverters and limiters, superconducting magnets, operation in the so-called "H-mode" island of increased stability,[221] and the compact tokamak, with the magnets on the inside of the vacuum chamber.[222][223]
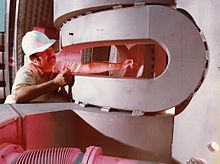
First inertial confinement experiments[edit]
Laser fusion was suggested in 1962 by scientists at Lawrence Livermore National Laboratory (LLNL), shortly after the invention of the laser in 1960. Inertial confinement fusion (using lasers) research began as early as 1965. Several laser systems were built at LLNL. These included the Argus, the Cyclops, the Janus, the long path, the Shiva laser, and the Nova.[224]
Laser advances included frequency-tripling crystals that transformed infrared laser beams into ultraviolet beams and "chirping", which changed a single wavelength into a full spectrum that could be amplified and then reconstituted into one frequency.[225] Laser research ate money as well, consuming over one billion dollars in the 1980s.[226]
1980s[edit]
The Tore Supra, JET, T-15, and JT-60 tokamaks were built in the 1980s.[227][228] In 1984, Martin Peng of ORNL proposed the spherical tokamak with a much smaller radius.[229] It used a single large conductor in the center, with magnets as half-rings off of this conductor. The aspect ratio fell to as low as 1.2.[230]:B247[231]:225 Peng's advocacy caught the interest of Derek Robinson, who built the Small Tight Aspect Ratio Tokamak, (START).[230]
1990s[edit]
In 1991, the Preliminary Tritium Experiment at the Joint European Torus achieved the world's first controlled release of fusion power.[232]
In 1996, Tore Supra created a plasma for two minutes with a current of almost 1 million amperes, totaling 280 MJ of injected and extracted energy.[233]
In 1997, JET produced a peak of 16.1 MW of fusion power (65% of heat to plasma[234]), with fusion power of over 10 MW sustained for over 0.5 sec.[235]
2000s[edit]

"Fast ignition"[236][237] saved power and moved ICF into the race for energy production.
In 2006, China's Experimental Advanced Superconducting Tokamak (EAST) test reactor was completed.[238] It was the first tokamak to use superconducting magnets to generate both toroidal and poloidal fields.
In March 2009, the laser-driven ICF NIF became operational.[239]
In the 2000s, privately backed fusion companies entered the race, including TAE Technologies,[240] General Fusion,[241][242] and Tokamak Energy.[243]
2010s[edit]
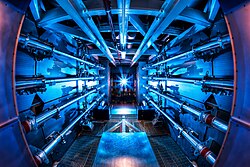


Private and public research accelerated in the 2010s. General Fusion developed plasma injector technology and Tri Alpha Energy tested its C-2U device.[244] The French Laser Mégajoule began operation. NIF achieved net energy gain[245] in 2013, as defined in the very limited sense as the hot spot at the core of the collapsed target, rather than the whole target.[246]
In 2014, Phoenix Nuclear Labs sold a high-yield neutron generator that could sustain 5×1011 deuterium fusion reactions per second over a 24-hour period.[247]
In 2015, MIT announced a tokamak it named the ARC fusion reactor, using rare-earth barium-copper oxide (REBCO) superconducting tapes to produce high-magnetic field coils that it claimed could produce comparable magnetic field strength in a smaller configuration than other designs.[248]
In October, researchers at the Max Planck Institute of Plasma Physics in Greifswald, Germany, completed building the largest stellarator to date, the Wendelstein 7-X (W7-X). The W7-X stellarator began Operational phase 1 (OP1.1) on 10 December 2015, successfully producing helium plasma.[249] The objective was to test vital systems and understand the machine's physics. By February 2016, hydrogen plasma was achieved, with temperatures reaching up to 100 million Kelvin. The initial tests used five graphite limiters. After over 2,000 pulses and achieving significant milestones, OP1.1 concluded on 10 March 2016. An upgrade followed, and OP1.2 in 2017 aimed to test an uncooled divertor. By June 2018, record temperatures were reached. W7-X concluded its first campaigns with limiter and island divertor tests, achieving notable advancements by the end of 2018.[250][251][252] It soon produced helium and hydrogen plasmas lasting up to 30 minutes.[253]
In 2017, Helion Energy's fifth-generation plasma machine went into operation.[254] The UK's Tokamak Energy's ST40 generated "first plasma".[255] The next year, Eni announced a $50 million investment in Commonwealth Fusion Systems, to attempt to commercialize MIT's ARC technology.[256][257][258][259]
2020s[edit]
In January 2021, SuperOx announced the commercialization of a new superconducting wire with more than 700 A/mm2 current capability.[260]
TAE Technologies announced results for its Norman device, holding a temperature of about 60 MK for 30 milliseconds, 8 and 10 times higher, respectively, than the company's previous devices.[261]
In October, Oxford-based First Light Fusion revealed its projectile fusion project, which fires an aluminum disc at a fusion target, accelerated by a 9 mega-amp electrical pulse, reaching speeds of 20 kilometres per second (12 mi/s). The resulting fusion generates neutrons whose energy is captured as heat.[262]
On November 8, in an invited talk to the 63rd Annual Meeting of the APS Division of Plasma Physics,[263] the National Ignition Facility claimed[264] to have triggered fusion ignition in the laboratory on August 8, 2021, for the first time in the 60+ year history of the ICF program.[265][266] The shot yielded 1.3 MJ of fusion energy, an over 8X improvement on tests done in spring of 2021.[264] NIF estimates that 230 kJ of energy reached the fuel capsule, which resulted in an almost 6-fold energy output from the capsule.[264] A researcher from Imperial College London stated that the majority of the field agreed that ignition had been demonstrated.[264]
In November 2021, Helion Energy reported receiving $500 million in Series E funding for its seventh-generation Polaris device, designed to demonstrate net electricity production, with an additional $1.7 billion of commitments tied to specific milestones,[267] while Commonwealth Fusion Systems raised an additional $1.8 billion in Series B funding to construct and operate its SPARC tokamak, the single largest investment in any private fusion company.[268]
In April 2022, First Light announced that their hypersonic projectile fusion prototype had produced neutrons compatible with fusion. Their technique electromagnetically fires projectiles at Mach 19 at a caged fuel pellet. The deuterium fuel is compressed at Mach 204, reaching pressure levels of 100 TPa.[269]
On December 13, 2022, the US Department of Energy reported that researchers at the National Ignition Facility had achieved a net energy gain from a fusion reaction. The reaction of hydrogen fuel at the facility produced about 3.15 MJ of energy while consuming 2.05 MJ of input. However, while the fusion reactions may have produced more than 3 megajoules of energy—more than was delivered to the target—NIF's 192 lasers consumed 322 MJ of grid energy in the conversion process.[1][2][270][271]
In May 2023, the United States Department of Energy (DOE) provided a grant of $46 million to eight companies across seven states to support fusion power plant design and research efforts. This funding, under the Milestone-Based Fusion Development Program, aligns with objectives to demonstrate pilot-scale fusion within a decade and to develop fusion as a carbon-neutral energy source by 2050. The granted companies are tasked with addressing the scientific and technical challenges to create viable fusion pilot plant designs in the next 5–10 years. The recipient firms include Commonwealth Fusion Systems, Focused Energy Inc., Princeton Stellarators Inc., Realta Fusion Inc., Tokamak Energy Inc., Type One Energy Group, Xcimer Energy Inc., and Zap Energy Inc.[272]
In December 2023, the largest and most advanced tokamak JT-60SA was inaugurated in Naka, Japan. The reactor is a joint project between Japan and the European Union. The reactor had achieved its first plasma in October 2023.[273] Subsequently, South Korea's fusion reactor project, the Korean Superconducting Tokamak Advanced Research, successfully operated for 102 seconds in a high-containment mode (H-mode) containing high ion temperatures of more than 100 million degrees in plasma tests conducted from December 2023 to February 2024.[274]
Records[edit]
Fusion records continue to advance:
Domain | Year | Record | Device | Notes |
---|---|---|---|---|
Plasma temperature | 2012 | 1.8×109 K | Focus-Fusion 1[275][276] | |
Fusion power | 1997 | 1.6×107 W | JET[277] | |
Tokamak fusion energy | 2023 | 6.9×107 J | JET[278] | |
ICF fusion energy | 2022 | 3.15×106 J | NIF[270] | Delivering 2.05 megajoules (MJ) of light energy to the target, resulting in 3.15 MJ of fusion energy output from appr. 400 MJ electric energy to drive the lasers. |
ICF shot rate | 2013 | Over 90K shots over 10 hours; Over 11 million pulse power shots at 10 Hz for over 12 days; | Electra laser at the Naval Research Laboratory[279] [280] | |
Plasma pressure | 2016 | 2.1×105 Pa | Alcator C-Mod[281] | |
Lawson criterion | 2013 | 1.53×1024 eV·s/m3 | JT-60[282][283] | |
Fusion energy gain factor | 2022 | 1.54 | NIF[270] | |
Confinement time (field reversed configuration) | 2016 | 3×10−1 s | Princeton Field Reversed Configuration[284] | Fusion was not observed. |
Confinement time (stellarator) | 2019 | >1×102 s | Wendelstein 7-X[285][286] | |
Confinement time (tokamak) | 2022 | >1×103 s | EAST[287] | |
Confinement time x temperature (tokamak) | 2021 | 1.2×1010 K·s | EAST[288] | |
Beta | 1998 | 0.4 | Small Tight Aspect Ratio Tokamak[289] | |
Temperature (compact spherical tokamak) | 2022 | 1×108 K | Tokamak Energy[290] | |
Temperature x time (tokamak) | 2021 | 3×109 K·s | KSTAR[291] |
See also[edit]
References[edit]
- ^ a b Chang, Kenneth (December 13, 2022). "Scientists Achieve Nuclear Fusion Breakthrough With Blast of 192 Lasers – The advancement by Lawrence Livermore National Laboratory researchers will be built on to further develop fusion energy research". The New York Times. Retrieved 2022-12-13.
- ^ a b "DOE National Laboratory Makes History by Achieving Fusion Ignition". US Department of Energy. December 13, 2022. Retrieved 2022-12-13.
- ^ Vogt, Adrienne; Hayes, Mike; Nilsen, Ella; Hammond, Elise (December 13, 2022). "December 13, 2022 US officials announce nuclear fusion breakthrough". CNN. Retrieved 2022-12-14.
- ^ Gardner, Timothy. "US scientists repeat fusion ignition breakthrough for 2nd time". Reuters. No. Dec 13, 2022. Retrieved 2024-02-13.
- ^ "Fuelling the fusion reaction". iter.org. ITER. Retrieved 2024-06-23.
- ^ Gan, Y; Hernandez, F; et, al (2017). "Thermal Discrete Element Analysis of EU Solid Breeder Blanket Subjected to Neutron Irradiation" (PDF). Fusion Science and Technology. 66 (1): 83–90. arXiv:1406.4199. doi:10.13182/FST13-727.
- ^ "Fission and fusion can yield energy". Hyperphysics.phy-astr.gsu.edu. Retrieved 2014-10-30.
- ^ a b c Miley, G. H.; Towner, H.; Ivich, N. (June 17, 1974). Fusion cross sections and reactivities (Technical Report). doi:10.2172/4014032. OSTI 4014032 – via Osti.gov.
- ^ a b c d e Lawson, J. D. (December 1, 1956). "Some Criteria for a Power Producing Thermonuclear Reactor". Proceedings of the Physical Society. Section B. 70 (1). IOP Publishing: 6–10. Bibcode:1957PPSB...70....6L. doi:10.1088/0370-1301/70/1/303. ISSN 0370-1301.
- ^ Wurzel, Samuel E., and Scott C. Hsu. "Progress toward fusion energy breakeven and gain as measured against the Lawson criterion." arXiv preprint arXiv:2105.10954 (2021).
- ^ "Lawson's three criteria". EFDA. February 25, 2013. Archived from the original on 2014-09-11. Retrieved 2014-08-24.
- ^ "Triple product". EFDA. June 20, 2014. Archived from the original on 2014-09-11. Retrieved 2014-08-24.
- ^ Chiocchio, Stefano. "ITER and the International ITER and the International Scientific Collaboration" (PDF).
- ^ "Laser Inertial Fusion Energy". Life.llnl.gov. Archived from the original on 2014-09-15. Retrieved 2014-08-24.
- ^ a b Barr, W. L.; Moir, R. W.; Hamilton, G. W. (1982). "Experimental results from a beam direct converter at 100 kV". Journal of Fusion Energy. 2 (2). Springer Science and Business Media LLC: 131–143. Bibcode:1982JFuE....2..131B. doi:10.1007/bf01054580. ISSN 0164-0313. S2CID 120604056.
- ^ Fitzpatrick, Richard (2014). Plasma physics: an introduction. Boca Raton, Florida: CRC Press, Taylor & Francis Group. ISBN 978-1466594265. OCLC 900866248.
- ^ Alfvén, H. (1942). "Existence of electromagnetic-hydrodynamic waves". Nature. 150 (3805): 405–406. Bibcode:1942Natur.150..405A. doi:10.1038/150405d0. S2CID 4072220.
- ^ Tuszewski, M. (1988). "Field reversed configurations". Nuclear Fusion (Submitted manuscript). 28 (11): 2033–2092. doi:10.1088/0029-5515/28/11/008. S2CID 122791237.
- ^ Sijoy, C. D.; Chaturvedi, Shashank (2012). "An Eulerian MHD model for the analysis of magnetic flux compression by expanding diamagnetic fusion plasma sphere". Fusion Engineering and Design. 87 (2): 104–117. Bibcode:2012FusED..87..104S. doi:10.1016/j.fusengdes.2011.10.012. ISSN 0920-3796.
- ^ Post, R. F. (1958). United Nations International Conference on the Peaceful Uses of Atomic Energy (ed.). Proceedings of the second United Nations International Conference on the Peaceful Uses of Atomic Energy held in Geneva 1 September – 13 September 1958. Vol. 32. Geneva, Switzerland: United Nations. OCLC 643589395.
- ^ "All-the-Worlds-Tokamaks". www.tokamak.info. Retrieved 2020-10-11.
- ^ "The first plasma: the Wendelstein 7-X fusion device is now in operation". www.ipp.mpg.de. Retrieved 2020-10-11.
- ^ Chandler, David (March 19, 2008). "MIT tests unique approach to fusion power". MIT News | Massachusetts Institute of Technology. Retrieved 2020-10-11.
- ^ a b Post, R. F. (January 1, 1970), "Mirror systems: fuel cycles, loss reduction and energy recovery", Nuclear fusion reactors, Conference Proceedings, Thomas Telford Publishing, pp. 99–111, doi:10.1680/nfr.44661, ISBN 978-0727744661, retrieved 2020-10-11
- ^ Berowitz, J. L; Grad, H.; Rubin, H. (1958). Proceedings of the second United Nations International Conference on the Peaceful Uses of Atomic Energy. Vol. 31. Geneva: United Nations. OCLC 840480538.
- ^ Bagryansky, P. A.; Shalashov, A. G.; Gospodchikov, E. D.; Lizunov, A. A.; Maximov, V. V.; Prikhodko, V. V.; Soldatkina, E. I.; Solomakhin, A. L.; Yakovlev, D. V. (May 18, 2015). "Threefold Increase of the Bulk Electron Temperature of Plasma Discharges in a Magnetic Mirror Device". Physical Review Letters. 114 (20): 205001. arXiv:1411.6288. Bibcode:2015PhRvL.114t5001B. doi:10.1103/physrevlett.114.205001. ISSN 0031-9007. PMID 26047233. S2CID 118484958.
- ^ Freidberg, Jeffrey P. (2007). Plasma Physics and Fusion Energy. Cambridge University Press. ISBN 978-0521851077.
- ^ Dolan, Thomas J., ed. (2013). Magnetic Fusion Technology. Lecture Notes in Energy. Vol. 19. London, England: Springer London. pp. 30–40. doi:10.1007/978-1-4471-5556-0. ISBN 978-1447155553. ISSN 2195-1284.
- ^ D. A. Sutherland, T. R. Jarboe et al., "The dynomak: An advanced spheromak reactor concept with imposed-dynamo current drive and next-generation nuclear power technologies", Fusion Engineering and Design, Volume 89, Issue 4, April 2014, pp. 412–425.
- ^ Jarboe, T. R., et al. "Spheromak formation by steady inductive helicity injection." Physical Review Letters 97.11 (2006): 115003
- ^ Jarboe, T. R., et al. "Recent results from the HIT-SI experiment." Nuclear Fusion 51.6 (2011): 063029
- ^ Nuckolls, John; Wood, Lowell; Thiessen, Albert; Zimmerman, George (1972). "Laser Compression of Matter to Super-High Densities: Thermonuclear (CTR) Applications". Nature. 239 (5368): 139–142. Bibcode:1972Natur.239..139N. doi:10.1038/239139a0. S2CID 45684425.
- ^ Turrell, Arthur (2021). How to Build a Star: the science of nuclear fusion and the quest to harness its power. Place of publication not identified: Weidenfeld & Nicolson. ISBN 978-1474611596. OCLC 1048447399.
- ^ Thio, Y. C. F. (April 1, 2008). "Status of the US program in magneto-inertial fusion". Journal of Physics: Conference Series. 112 (4). IOP Publishing: 042084. Bibcode:2008JPhCS.112d2084T. doi:10.1088/1742-6596/112/4/042084. ISSN 1742-6596.
- ^ Sharp, W. M.; et al. (2011). Inertial Fusion Driven by Intense Heavy-Ion Beams (PDF). Proceedings of 2011 Particle Accelerator Conference. New York, New York, USA. p. 1386. Archived from the original (PDF) on 2017-11-26. Retrieved 2019-08-03.
- ^ a b Seife, Charles (2008). Sun in a bottle: the strange history of fusion and the science of wishful thinking. New York: Viking. ISBN 978-0670020331. OCLC 213765956.
- ^ a b c Phillips, James (1983). "Magnetic Fusion". Los Alamos Science: 64–67. Archived from the original on 2016-12-23. Retrieved 2013-04-04.
- ^ "Flow Z-Pinch Experiments". Aeronautics and Astronautics. November 7, 2014. Retrieved 2020-10-11.
- ^ "Zap Energy". Zap Energy. Archived from the original on 2020-02-13. Retrieved 2020-02-13.
- ^ "Board of Directors". ZAP ENERGY. Retrieved 2020-09-08.
- ^ "Chevron announces investment in nuclear fusion start-up Zap Energy". Power Technology | Energy News and Market Analysis. August 13, 2020. Retrieved 2020-09-08.
- ^ Srivastava, Krishna M.; Vyas, D. N. (1982). "Non-linear analysis of the stability of the Screw Pinch". Astrophysics and Space Science. 86 (1). Springer Nature: 71–89. Bibcode:1982Ap&SS..86...71S. doi:10.1007/bf00651831. ISSN 0004-640X. S2CID 121575638.
- ^ Rider, Todd H. (1995). "A general critique of inertial-electrostatic confinement fusion systems". Physics of Plasmas. 2 (6). AIP Publishing: 1853–1872. Bibcode:1995PhPl....2.1853R. doi:10.1063/1.871273. hdl:1721.1/29869. ISSN 1070-664X. S2CID 12336904.
- ^ Clynes, Tom (February 14, 2012). "The Boy Who Played With Fusion". Popular Science. Retrieved 2019-08-03.
- ^ US patent 5,160,695, Robert W. Bussard, "Method and apparatus for creating and controlling nuclear fusion reactions", issued 1992-11-03
- ^ Taccetti, J. M.; Intrator, T. P.; Wurden, G. A.; Zhang, S. Y.; Aragonez, R.; Assmus, P. N.; Bass, C. M.; Carey, C.; deVries, S. A.; Fienup, W. J.; Furno, I. (September 25, 2003). "FRX-L: A field-reversed configuration plasma injector for magnetized target fusion". Review of Scientific Instruments. 74 (10): 4314–4323. Bibcode:2003RScI...74.4314T. doi:10.1063/1.1606534. ISSN 0034-6748.
- ^ Hsu, S. C.; Awe, T. J.; Brockington, S.; Case, A.; Cassibry, J. T.; Kagan, G.; Messer, S. J.; Stanic, M.; Tang, X.; Welch, D. R.; Witherspoon, F. D. (2012). "Spherically Imploding Plasma Liners as a Standoff Driver for Magnetoinertial Fusion". IEEE Transactions on Plasma Science. 40 (5): 1287–1298. Bibcode:2012ITPS...40.1287H. doi:10.1109/TPS.2012.2186829. ISSN 1939-9375. S2CID 32998378.
- ^ a b Clynes, Tom (2020). "5 Big ideas for fusion power: Startups, universities, and major companies are vying to commercialize a nuclear fusion reactor". IEEE Spectrum. 57 (2): 30–37. doi:10.1109/MSPEC.2020.8976899. ISSN 0018-9235. S2CID 211059641.
- ^ Nagamine 2003.
- ^ Nagamine, K. (2007). Introductory muon science. Cambridge, England: Cambridge University Press. ISBN 978-0521038201. OCLC 124025585.
- ^ Baramsai, Bayardadrakh; Benyo, Theresa; Forsley, Lawrence; Steinetz, Bruce (February 27, 2022). "NASA's New Shortcut to Fusion Power". IEEE Spectrum.
- ^ Steinetz, Bruce M.; Benyo, Theresa L.; Chait, Arnon; Hendricks, Robert C.; Forsley, Lawrence P.; Baramsai, Bayarbadrakh; Ugorowski, Philip B.; Becks, Michael D.; Pines, Vladimir; Pines, Marianna; Martin, Richard E.; Penney, Nicholas; Fralick, Gustave C.; Sandifer, Carl E. (April 20, 2020). "Novel nuclear reactions observed in bremsstrahlung-irradiated deuterated metals". Physical Review C. 101 (4): 044610. Bibcode:2020PhRvC.101d4610S. doi:10.1103/physrevc.101.044610. S2CID 219083603 – via APS.
- ^ "Plasma Physics". Government Reports Announcements. 72: 194. 1972.
- ^ Katwala, Amit (February 16, 2022). "DeepMind Has Trained an AI to Control Nuclear Fusion". Wired. ISSN 1059-1028. Retrieved 2022-02-17.
- ^ Katwala, Amit. "DeepMind Has Trained an AI to Control Nuclear Fusion". Wired.
- ^ Miley, George H. (2013). Inertial electrostatic confinement (IEC) fusion : fundamentals and applications. Murali, S. Krupakar. Dordrecht: Springer. ISBN 978-1461493389. OCLC 878605320.
- ^ Kunkel, W. B. (1981). "Neutral-beam injection". In Teller, E. (ed.). Fusion. Lawrence Livermore National Laboratory. ISBN 978-0126852417.
- ^ Erckmann, V; Gasparino, U (December 1, 1994). "Electron cyclotron resonance heating and current drive in toroidal fusion plasmas". Plasma Physics and Controlled Fusion. 36 (12): 1869–1962. Bibcode:1994PPCF...36.1869E. doi:10.1088/0741-3335/36/12/001. ISSN 0741-3335. S2CID 250897078.
- ^ Ono, Y.; Tanabe, H.; Yamada, T.; Gi, K.; Watanabe, T.; Ii, T.; Gryaznevich, M.; Scannell, R.; Conway, N.; Crowley, B.; Michael, C. (May 1, 2015). "High power heating of magnetic reconnection in merging tokamak experiments". Physics of Plasmas. 22 (5): 055708. Bibcode:2015PhPl...22e5708O. doi:10.1063/1.4920944. hdl:1885/28549. ISSN 1070-664X.
- ^ Yamada, M.; Chen, L.-J.; Yoo, J.; Wang, S.; Fox, W.; Jara-Almonte, J.; Ji, H.; Daughton, W.; Le, A.; Burch, J.; Giles, B. (December 6, 2018). "The two-fluid dynamics and energetics of the asymmetric magnetic reconnection in laboratory and space plasmas". Nature Communications. 9 (1): 5223. Bibcode:2018NatCo...9.5223Y. doi:10.1038/s41467-018-07680-2. ISSN 2041-1723. PMC 6283883. PMID 30523290.
- ^ McGuire, Thomas. Heating Plasma for Fusion Power Using Magnetic Field Oscillations. Baker Botts LLP, assignee. Issued: 4/2/14, Patent 14/243,447. N.d. Print.
- ^ "Towards a fusion reactor", Nuclear Fusion, IOP Publishing Ltd, 2002, doi:10.1887/0750307056/b888c9, ISBN 0750307056, retrieved 2021-12-12
- ^ Pearson, Richard J; Takeda, Shutaro (2020), "Review of approaches to fusion energy", Commercialising Fusion Energy, IOP Publishing, doi:10.1088/978-0-7503-2719-0ch2, ISBN 978-0750327190, S2CID 234561187, retrieved 2021-12-12
- ^ Labik, George; Brown, Tom; Johnson, Dave; Pomphrey, Neil; Stratton, Brentley; Viola, Michael; Zarnstorff, Michael; Duco, Mike; Edwards, John; Cole, Mike; Lazarus, Ed (2007). "National Compact Stellarator Experiment Vacuum Vessel External Flux Loops Design and Installation". 2007 IEEE 22nd Symposium on Fusion Engineering. pp. 1–3. doi:10.1109/FUSION.2007.4337935. ISBN 978-1424411931. S2CID 9298179.
- ^ Park, Jaeyoung; Krall, Nicholas A.; Sieck, Paul E.; Offermann, Dustin T.; Skillicorn, Michael; Sanchez, Andrew; Davis, Kevin; Alderson, Eric; Lapenta, Giovanni (June 1, 2014). "High Energy Electron Confinement in a Magnetic Cusp Configuration". Physical Review X. 5 (2): 021024. arXiv:1406.0133. Bibcode:2015PhRvX...5b1024P. doi:10.1103/PhysRevX.5.021024. S2CID 118478508.
- ^ Mott-Smith, H. M.; Langmuir, Irving (September 1, 1926). "The Theory of Collectors in Gaseous Discharges". Physical Review. 28 (4). American Physical Society (APS): 727–763. Bibcode:1926PhRv...28..727M. doi:10.1103/physrev.28.727. ISSN 0031-899X.
- ^ Esarey, Eric; Ride, Sally K.; Sprangle, Phillip (September 1, 1993). "Nonlinear Thomson scattering of intense laser pulses from beams and plasmas". Physical Review E. 48 (4). American Physical Society (APS): 3003–3021. Bibcode:1993PhRvE..48.3003E. doi:10.1103/physreve.48.3003. ISSN 1063-651X. PMID 9960936.
- ^ Kantor, M. Yu; Donné, A. J. H.; Jaspers, R.; van der Meiden, H. J. (February 26, 2009). "Thomson scattering system on the TEXTOR tokamak using a multi-pass laser beam configuration". Plasma Physics and Controlled Fusion. 51 (5): 055002. Bibcode:2009PPCF...51e5002K. doi:10.1088/0741-3335/51/5/055002. ISSN 0741-3335. S2CID 123495440.
- ^ Tsoulfanidis, Nicholas (1995). Measurement and detection of radiation. Library Genesis. Washington, DC : Taylor & Francis. ISBN 978-1560323174.
- ^ Knoll, Glenn F. (2010). Radiation detection and measurement (4th ed.). Hoboken, NJ: John Wiley. ISBN 978-0470131480. OCLC 612350364.
- ^ Larmor, Joseph (January 1, 1897). "IX. A dynamical theory of the electric and luminiferous medium. Part III. relations with material media". Philosophical Transactions of the Royal Society of London. Series A, Containing Papers of a Mathematical or Physical Character. 190: 205–300. Bibcode:1897RSPTA.190..205L. doi:10.1098/rsta.1897.0020.
- ^ Stott PE, Gorini G, Prandoni P, Sindoni E, eds. (1998). Diagnostics for experimental thermonuclear fusion reactors 2. New York: Springer. ISBN 978-1461553533. OCLC 828735433.
- ^ Ishiyama, Shintaro; Muto, Yasushi; Kato, Yasuyoshi; Nishio, Satoshi; Hayashi, Takumi; Nomoto, Yasunobu (March 1, 2008). "Study of steam, helium and supercritical CO2 turbine power generations in prototype fusion power reactor". Progress in Nuclear Energy. Innovative Nuclear Energy Systems for Sustainable Development of the World. Proceedings of the Second COE-INES International Symposium, INES-2, November 26–30, 2006, Yokohama, Japan. 50 (2): 325–332. doi:10.1016/j.pnucene.2007.11.078. ISSN 0149-1970.
- ^ Anklam, T.; Simon, A. J.; Powers, S.; Meier, W. R. (December 2, 2010). "LIFE: The Case for Early Commercialization of Fusion Energy" (PDF). Lawrence Livermore National Laboratory, LLNL-JRNL-463536. Archived from the original (PDF) on 2015-09-04. Retrieved 2014-10-30.
- ^ Hanaor, D. A. H.; Kolb, M. H. H.; Gan, Y.; Kamlah, M.; Knitter, R. (2014). "Solution based synthesis of mixed-phase materials in the Li2TiO3-Li4SiO4 system". Journal of Nuclear Materials. 456: 151–161. arXiv:1410.7128. Bibcode:2015JNuM..456..151H. doi:10.1016/j.jnucmat.2014.09.028. S2CID 94426898.
- ^ Barr, William L.; Moir, Ralph W. (January 1, 1983). "Test Results on Plasma Direct Converters". Nuclear Technology – Fusion. 3 (1): 98–111. Bibcode:1983NucTF...3...98B. doi:10.13182/FST83-A20820. ISSN 0272-3921.
- ^ Booth, William (October 9, 1987). "Fusion's $372-Million Mothball". Science. 238 (4824): 152–155. Bibcode:1987Sci...238..152B. doi:10.1126/science.238.4824.152. PMID 17800453.
- ^ Grad, Harold (2016). Containment in cusped plasma systems (classic reprint). Forgotten Books. ISBN 978-1333477035. OCLC 980257709.
- ^ Lee, Chris (June 22, 2015). "Magnetic mirror holds promise for fusion". Ars Technica. Retrieved 2020-10-11.
- ^ a b Pfalzner, Susanne (2006). An introduction to inertial confinement fusion. New York: Taylor & Francis/CRC Press. ISBN 1420011847. OCLC 72564680.
- ^ Thorson, Timothy A. (1996). Ion flow and fusion reactivity characterization of a spherically convergent ion focus. University of Wisconsin, Madison.
- ^ Barnes, D. C.; Nebel, R. A. (July 1998). "Stable, thermal equilibrium, large-amplitude, spherical plasma oscillations in electrostatic confinement devices". Physics of Plasmas. 5 (7): 2498–2503. Bibcode:1998PhPl....5.2498B. doi:10.1063/1.872933. ISSN 1070-664X.
- ^ Hedditch, John; Bowden-Reid, Richard; Khachan, Joe (October 2015). "Fusion in a magnetically-shielded-grid inertial electrostatic confinement device". Physics of Plasmas. 22 (10): 102705. arXiv:1510.01788. Bibcode:2015PhPl...22j2705H. doi:10.1063/1.4933213. ISSN 1070-664X.
- ^ Carr, M.; Khachan, J. (2013). "A biased probe analysis of potential well formation in an electron only, low beta Polywell magnetic field". Physics of Plasmas. 20 (5): 052504. Bibcode:2013PhPl...20e2504C. doi:10.1063/1.4804279.
- ^ Sieckand, Paul; Volberg, Randall (2017). Fusion One Corporation (PDF). Fusion One Corporation.
- ^ Atzeni, Stefano; Meyer-ter-Vehn, Jürgen (June 3, 2004). The Physics of Inertial Fusion: BeamPlasma Interaction, Hydrodynamics, Hot Dense Matter. OUP Oxford. pp. 12–13. ISBN 978-0191524059.
- ^ Velarde, Guillermo; Martínez-Val, José María; Ronen, Yigal (1993). Nuclear fusion by inertial confinement: a comprehensive treatise. Boca Raton; Ann Arbor; London: CRC Press. ISBN 978-0849369261. OCLC 468393053.
- ^ Iiyoshi, A; Momota, H.; Motojima, O.; et al. (October 1993). "Innovative Energy Production in Fusion Reactors". National Institute for Fusion Science NIFS: 2–3. Bibcode:1993iepf.rept.....I. Archived from the original on 2015-09-04. Retrieved 2012-02-14.
- ^ "Nuclear Fusion : WNA – World Nuclear Association". www.world-nuclear.org. Retrieved 2020-10-11.
- ^ Rolfe, A. C. (1999). "Remote Handling JET Experience" (PDF). Nuclear Energy. 38 (5): 6. ISSN 0140-4067. Retrieved 2012-04-10.
- ^ Sawan, M. E.; Zinkle, S. J.; Sheffield, J. (2002). "Impact of tritium removal and He-3 recycling on structure damage parameters in a D–D fusion system". Fusion Engineering and Design. 61–62: 561–567. Bibcode:2002FusED..61..561S. doi:10.1016/s0920-3796(02)00104-7. ISSN 0920-3796.
- ^ J. Kesner, D. Garnier, A. Hansen, M. Mauel, and L. Bromberg, Nucl Fusion 2004; 44, 193
- ^ a b Nevins, W. M. (March 1, 1998). "A Review of Confinement Requirements for Advanced Fuels". Journal of Fusion Energy. 17 (1): 25–32. Bibcode:1998JFuE...17...25N. doi:10.1023/A:1022513215080. ISSN 1572-9591. S2CID 118229833.
- ^ von Möllendorff, Ulrich; Goel, Balbir, eds. (1989). Emerging nuclear energy systems 1989: proceedings of the Fifth International Conference on Emerging Nuclear Energy Systems, Karlsruhe, F.R. Germany, July 3–6, 1989. Singapore: World Scientific. ISBN 981-0200102. OCLC 20693180.
- ^ Feldbacher, Rainer; Heindler, Manfred (1988). "Basic cross section data for aneutronic reactor". Nuclear Instruments and Methods in Physics Research Section A: Accelerators, Spectrometers, Detectors and Associated Equipment. 271 (1): 55–64. Bibcode:1988NIMPA.271...55F. doi:10.1016/0168-9002(88)91125-4. ISSN 0168-9002.
- ^ "Nuclear Fusion: Laser-Beam Experiment Yields Exciting Results". LiveScience.com. October 8, 2013.
- ^ "Record proton-boron fusion rate achieved – FuseNet". www.fusenet.eu. Archived from the original on 2014-12-02. Retrieved 2014-11-26.
- ^ a b c d e Roberts, J. T. Adrian (1981). Structural Materials in Nuclear Power Systems. Boston, MA: Springer US. ISBN 978-1468471960. OCLC 853261260.
- ^ "Roadmap highlights materials route to fusion". The Engineer. September 9, 2021. Retrieved 2021-09-17.
- ^ Klueh, R. L. "Metals in the nuclear-fusion environment". Materials Engineering. 99: 39–42.
- ^ Založnik, Anže (2016). Interaction of atomic hydrogen with materials used for plasma-facing wall in fusion devices (Doctorate). Ljubljana: [A. Založnik]. OCLC 958140759.
- ^ McCracken, G. M. (1997). "Plasma surface interactions in controlled fusion devices". Nuclear Fusion. 37 (3): 427–429. doi:10.1088/0029-5515/37/3/413. ISSN 0029-5515. S2CID 250776874.
- ^ Mioduszewski, Peter (2000), "Hydrogen Recycling and Wall Equilibration in Fusion Devices", Hydrogen Recycling at Plasma Facing Materials, Dordrecht: Springer Netherlands, pp. 195–201, doi:10.1007/978-94-011-4331-8_23, ISBN 978-0792366300, retrieved 2020-10-13
- ^ a b c Nemanič, Vincenc (2019). "Hydrogen permeation barriers: Basic requirements, materials selection, deposition methods, and quality evaluation". Nuclear Materials and Energy. 19: 451–457. Bibcode:2019NMEne..19..451N. doi:10.1016/j.nme.2019.04.001. ISSN 2352-1791.
- ^ "American Elements Creates Detection Window for EPFL Fusion Reactor". American Elements. Retrieved 2023-02-16.
- ^ Molodyk, A., et al. "Development and large volume production of extremely high current density YBa2Cu3O7 superconducting wires for fusion." Scientific Reports 11.1 (2021): 1–11.
- ^ a b "Thermal response of nanostructured tungsten". Shin Kajita, et al., January 2014, Nuclear Fusion 54 (2014) 033005 (10 pp.)
- ^ Brezɩnsek, S.; et al. (December 2, 2021). "Plasma–surface interaction in the stellarator W7-X: Conclusions drawn from operation with graphite plasma-facing components". Nuclear Fusion. 62 (1): 016006. doi:10.1088/1741-4326/ac3508. S2CID 240484560.
- ^ Hoedl, Seth A. (2022). "Achieving a social license for fusion energy". Physics of Plasmas. 29 (9): 092506. Bibcode:2022PhPl...29i2506H. doi:10.1063/5.0091054. ISSN 1070-664X. S2CID 252454077.
- ^ a b c McCracken, Garry; Stott, Peter (2012). Fusion: The Energy of the Universe. Academic Press. pp. 198–199. ISBN 978-0123846563. Retrieved 2012-08-18.
- ^ Dulon, Krista (2012). "Who is afraid of ITER?". iter.org. Archived from the original on 2012-11-30. Retrieved 2012-08-18.
- ^ Angelo, Joseph A. (2004). Nuclear Technology. Greenwood Publishing Group. p. 474. ISBN 978-1573563369. Retrieved 2012-08-18.
- ^ a b Brunelli, B.; Knoepfel, Heinz, eds. (1990). Safety, environmental impact, and economic prospects of nuclear fusion. New York: Plenum Press. ISBN 978-1461306191. OCLC 555791436.
- ^ a b Hamacher, T.; Bradshaw, A. M. (October 2001). "Fusion as a Future Power Source: Recent Achievements and Prospects" (PDF). World Energy Council. Archived from the original (PDF) on 2004-05-06.
- ^ Interim Summary Report on the Analysis of the 19 September 2008 Incident at the LHC (PDF). CERN. 2008.
- ^ Peterson, Tom (November 2008). "Explain it in 60 seconds: Magnet Quench". Symmetry Magazine. Fermilab/SLAC. Retrieved 2013-02-15.
- ^ Petrangeli, Gianni (2006). Nuclear Safety. Butterworth-Heinemann. p. 430. ISBN 978-0750667234.
- ^ a b Claessens, Michel (2019). ITER: the giant fusion reactor: bringing a sun to Earth. Cham: Springer. ISBN 978-3030275815. OCLC 1124925935.
- ^ a b Gonzalez de Vicente, Sehila M.; Smith, Nicholas A.; El-Guebaly, Laila; Ciattaglia, Sergio; Di Pace, Luigi; Gilbert, Mark; Mandoki, Robert; Rosanvallon, Sandrine; Someya, Youji; Tobita, Kenji; Torcy, David (August 1, 2022). "Overview on the management of radioactive waste from fusion facilities: ITER, demonstration machines and power plants". Nuclear Fusion. 62 (8): 085001. Bibcode:2022NucFu..62h5001G. doi:10.1088/1741-4326/ac62f7. ISSN 0029-5515. S2CID 247920590.
- ^ Harms, A. A.; Schoepf, Klaus F.; Kingdon, David Ross (2000). Principles of Fusion Energy: An Introduction to Fusion Energy for Students of Science and Engineering. World Scientific. ISBN 978-9812380333.
- ^ Carayannis, Elias G.; Draper, John; Iftimie, Ion A. (2020). "Nuclear Fusion Diffusion: Theory, Policy, Practice, and Politics Perspectives". IEEE Transactions on Engineering Management. 69 (4): 1237–1251. doi:10.1109/TEM.2020.2982101. ISSN 1558-0040. S2CID 219001461.
- ^ Markandya, Anil; Wilkinson, Paul (2007). "Electricity generation and health". The Lancet. 370 (9591): 979–990. doi:10.1016/S0140-6736(07)61253-7. PMID 17876910. S2CID 25504602. Retrieved 2018-02-21.
- ^ Nicholas, T. E. G.; Davis, T. P.; Federici, F.; Leland, J.; Patel, B. S.; Vincent, C.; Ward, S. H. (February 1, 2021). "Re-examining the role of nuclear fusion in a renewables-based energy mix". Energy Policy. 149: 112043. arXiv:2101.05727. Bibcode:2021EnPol.14912043N. doi:10.1016/j.enpol.2020.112043. ISSN 0301-4215. S2CID 230570595.
- ^ Cheng, E. T.; Muroga, Takeo (2001). "Reuse of Vanadium Alloys in Power Reactors". Fusion Technology. 39 (2P2): 981–985. Bibcode:2001FuTec..39..981C. doi:10.13182/fst01-a11963369. ISSN 0748-1896. S2CID 124455585.
- ^ Streckert, H. H.; Schultz, K. R.; Sager, G. T.; Kantncr, R. D. (December 1, 1996). "Conceptual Design of Low Activation Target Chamber and Components for the National Ignition Facility". Fusion Technology. 30 (3P2A): 448–451. Bibcode:1996FuTec..30..448S. CiteSeerX 10.1.1.582.8236. doi:10.13182/FST96-A11962981. ISSN 0748-1896.
- ^ a b c d R. J. Goldston, A. Glaser, A. F. Ross: "Proliferation Risks of Fusion Energy: Clandestine Production, Covert Production, and Breakout" Archived 2014-02-27 at the Wayback Machine;9th IAEA Technical Meeting on Fusion Power Plant Safety (accessible at no cost, 2013) and Glaser, A.; Goldston, R. J. (2012). "Proliferation risks of magnetic fusion energy: Clandestine production, covert production and breakout". Nuclear Fusion. 52 (4). 043004. Bibcode:2012NucFu..52d3004G. doi:10.1088/0029-5515/52/4/043004. S2CID 73700489.
- ^ a b Englert, Matthias; Franceschini, Giorgio; Liebert, Wolfgang (2011). Strong Neutron Sources – How to cope with weapon material production capabilities of fusion and spallation neutron sources? (PDF). 7th INMM/Esarda Workshop, Aix-en-Provence. Archived from the original (PDF) on 2014-02-24.
- ^ "Energy for Future Centuries" (PDF). Archived from the original (PDF) on 2011-07-27. Retrieved 2013-06-22.
- ^ Christian, Eric; et al. "Cosmicopia". NASA. Archived from the original on 2011-11-06. Retrieved 2009-03-20.
- ^ Fusion For Energy. "Fusion For Energy – Bringing the power of the sun to earth". f4e.europa.eu. Archived from the original on 2019-11-29. Retrieved 2020-07-17.
- ^ "ITER governing council pushes schedule back five years and trims budget". Physics Today. 2016. doi:10.1063/pt.5.029905. ISSN 1945-0699.
- ^ "ITER disputes DOE's cost estimate of fusion project". Physics Today. 2018. doi:10.1063/PT.6.2.20180416a.
- ^ "The Sixth Framework Programme in brief" (PDF). ec.europa.eu. Retrieved 2014-10-30.
- ^ Margraf, Rachel. "A Brief History of U.S. Funding of Fusion Energy". Retrieved 2021-07-21.
- ^ DOE/CF-0167 – Department of Energy FY 2021 Congressional Budget Request, Budget in Brief, February 2020. https://www.energy.gov/sites/default/files/2020/02/f72/doe-fy2021-budget-in-brief_0.pdf Archived 2021-07-18 at the Wayback Machine
- ^ Nuttall, William J., ed. (2020). Commercialising fusion energy : how small businesses are transforming big science. Institute of Physics. ISBN 978-0750327176. OCLC 1230513895.
- ^ Fusion Energy Sciences Advisory Committee (2021). Powering the Future: Fusion & Plasmas (PDF). Washington: Department of Energy Fusion Energy Sciences. pp. ii.
- ^ Helman, Christopher. "Fueled By Billionaire Dollars, Nuclear Fusion Enters A New Age". Forbes. Retrieved 2022-01-14.
- ^ a b c Windridge, Melanie. "The New Space Race Is Fusion Energy". Forbes. Retrieved 2020-10-10.
- ^ Pearson, Richard J.; Takeda, Shutaro (2020), "Review of approaches to fusion energy", Commercialising Fusion Energy, IOP Publishing, doi:10.1088/978-0-7503-2719-0ch2, ISBN 978-0750327190, S2CID 234561187, retrieved 2021-12-13
- ^ Pearson, Richard J.; Nuttall, William J. (2020), "Pioneers of commercial fusion", Commercialising Fusion Energy, IOP Publishing, doi:10.1088/978-0-7503-2719-0ch7, ISBN 978-0750327190, S2CID 234528929, retrieved 2021-12-13
- ^ a b Carayannis, Elias G.; Draper, John; Iftimie, Ion A. (2020). "Nuclear Fusion Diffusion: Theory, Policy, Practice, and Politics Perspectives". IEEE Transactions on Engineering Management. 69 (4): 1237–1251. doi:10.1109/TEM.2020.2982101. ISSN 0018-9391. S2CID 219001461.
- ^ a b "White House Sets Sights on Commercial Fusion Energy". www.aip.org. April 25, 2022. Retrieved 2022-05-03.
- ^ a b Lee, Sing; Saw, Sor Heoh. "Nuclear Fusion Energy – Mankind's Giant Step Forward" (PDF). HPlasmafocus.net. Retrieved 2014-10-30.
- ^ Kessel, C. E.; Blanchard, J. P.; Davis, A.; El-Guebaly, L.; Ghoniem, N.; Humrickhouse, P. W.; Malang, S.; Merrill, B. J.; Morley, N. B.; Neilson, G. H.; Rensink, M. E. (September 1, 2015). "The Fusion Nuclear Science Facility, the Critical Step in the Pathway to Fusion Energy". Fusion Science and Technology. 68 (2): 225–236. Bibcode:2015FuST...68..225K. doi:10.13182/FST14-953. ISSN 1536-1055. OSTI 1811772. S2CID 117842168.
- ^ a b Menard, J. E.; Brown, T.; El-Guebaly, L.; Boyer, M.; Canik, J.; Colling, B.; Raman, R.; Wang, Z.; Zhai, Y.; Buxton, P.; Covele, B. (October 1, 2016). "Fusion nuclear science facilities and pilot plants based on the spherical tokamak". Nuclear Fusion. 56 (10): 106023. Bibcode:2016NucFu..56j6023M. doi:10.1088/0029-5515/56/10/106023. ISSN 0029-5515. S2CID 125184562.
- ^ Cardozo, N. J. Lopes (February 4, 2019). "Economic aspects of the deployment of fusion energy: the valley of death and the innovation cycle". Philosophical Transactions of the Royal Society A: Mathematical, Physical and Engineering Sciences. 377 (2141): 20170444. Bibcode:2019RSPTA.37770444C. doi:10.1098/rsta.2017.0444. ISSN 1364-503X. PMID 30967058. S2CID 106411210.
- ^ Surrey, E. (February 4, 2019). "Engineering challenges for accelerated fusion demonstrators". Philosophical Transactions of the Royal Society A: Mathematical, Physical and Engineering Sciences. 377 (2141): 20170442. Bibcode:2019RSPTA.37770442S. doi:10.1098/rsta.2017.0442. ISSN 1364-503X. PMC 6365852. PMID 30967054.
- ^ The Fusion Industry Supply Chain: Opportunities and Challenges. Washington, DC: Fusion Industry Association. 2023.
- ^ Banacloche, Santacruz; Gamarra, Ana R.; Lechon, Yolanda; Bustreo, Chiara (October 15, 2020). "Socioeconomic and environmental impacts of bringing the sun to earth: A sustainability analysis of a fusion power plant deployment". Energy. 209: 118460. Bibcode:2020Ene...20918460B. doi:10.1016/j.energy.2020.118460. ISSN 0360-5442. S2CID 224952718.
- ^ Koepke, M. E. (January 25, 2021). "Factors influencing the commercialization of inertial fusion energy". Philosophical Transactions of the Royal Society A: Mathematical, Physical and Engineering Sciences. 379 (2189): 20200020. Bibcode:2021RSPTA.37900020K. doi:10.1098/rsta.2020.0020. ISSN 1364-503X. PMC 7741007. PMID 33280558.
- ^ Menard, J. E.; Bromberg, L.; Brown, T.; Burgess, T.; Dix, D.; El-Guebaly, L.; Gerrity, T.; Goldston, R. J.; Hawryluk, R. J.; Kastner, R.; Kessel, C. (October 1, 2011). "Prospects for pilot plants based on the tokamak, spherical tokamak and stellarator". Nuclear Fusion. 51 (10): 103014. Bibcode:2011NucFu..51j3014M. doi:10.1088/0029-5515/51/10/103014. ISSN 0029-5515. S2CID 55781189.
- ^ a b Hiwatari, Ryoji; Goto, Takuya (March 19, 2019). "Assessment on Tokamak Fusion Power Plant to Contribute to Global Climate Stabilization in the Framework of Paris Agreement". Plasma and Fusion Research. 14: 1305047. Bibcode:2019PFR....1405047H. doi:10.1585/pfr.14.1305047. ISSN 1880-6821.
- ^ National Academies of Sciences, Engineering, and Medicine (U.S.). Committee on a Strategic Plan for U.S. Burning Plasma Research. Final report of the Committee on a Strategic Plan for U.S. Burning Plasma Research. Washington, DC. ISBN 978-0309487443. OCLC 1104084761.
- ^ A Community Plan for Fusion Energy and Discovery Plasma Sciences. Washington, DC: American Physical Society Division of Plasma Physics Community Planning Process. 2020.
- ^ "US Plasma Science Strategic Planning Reaches Pivotal Phase". www.aip.org. April 7, 2020. Retrieved 2020-10-08.
- ^ Asmundssom, Jon; Wade, Will. "Nuclear Fusion Could Rescue the Planet from Climate Catastrophe". Bloomberg. Retrieved 2020-09-21.
- ^ Michaels, Daniel (February 6, 2020). "Fusion Startups Step In to Realize Decades-Old Clean Power Dream". The Wall Street Journal. ISSN 0099-9660. Retrieved 2020-10-08.
- ^ a b c d Handley, Malcolm C.; Slesinski, Daniel; Hsu, Scott C. (July 10, 2021). "Potential Early Markets for Fusion Energy". Journal of Fusion Energy. 40 (2): 18. arXiv:2101.09150. doi:10.1007/s10894-021-00306-4. ISSN 0164-0313. S2CID 231693147.
- ^ Ball, Philip (November 17, 2021). "The chase for fusion energy". Nature. 599 (7885): 352–366. doi:10.1038/d41586-021-03401-w. PMID 34789909. S2CID 244346561.
- ^ "A Historic Decision: To Demonstrate Practical Fusion at Culham". General Fusion. June 16, 2021. Retrieved 2021-06-18.
- ^ Holland, Andrew (July 15, 2021). "Congress Would Fund Fusion Cost-Share Program in Committee-Passed Appropriations Bill". Fusion Industry Assn. Archived from the original on 2023-04-20. Retrieved 2021-07-16.
- ^ Sailer, Sandy (May 31, 2023). "Department of Energy Announces Milestone Public-Private Partnership Awards". Fusion Industry Association. Retrieved 2023-06-01.
- ^ Hsu, Scott C. (May 5, 2023). "U.S. Fusion Energy Development via Public-Private Partnerships". Journal of Fusion Energy. 42 (1). doi:10.1007/s10894-023-00357-9. ISSN 0164-0313. S2CID 258489130.
- ^ Spangher, Lucas; Vitter, J. Scott; Umstattd, Ryan (2019). "Characterizing fusion market entry via an agent-based power plant fleet model". Energy Strategy Reviews. 26: 100404. Bibcode:2019EneSR..2600404S. doi:10.1016/j.esr.2019.100404. ISSN 2211-467X.
- ^ "Global Energy Perspectives 2019". Energy Insights- Mckinsey.
- ^ a b c d e f g Nicholas, T. E. G.; Davis, T. P.; Federici, F.; Leland, J. E.; Patel, B. S.; Vincent, C.; Ward, S. H. (February 2021). "Re-examining the Role of Nuclear Fusion in a Renewables-Based Energy Mix". Energy Policy. 149: 112043. arXiv:2101.05727. Bibcode:2021EnPol.14912043N. doi:10.1016/j.enpol.2020.112043. S2CID 230570595.
- ^ "2023 Levelized Cost Of Energy+". Lazard. April 12, 2023. p. 9. Archived from the original on 2023-08-27. (Download link labeled "Lazard's LCOE+ (April 2023) (1) PDF—1MB")
- ^ Entler, Slavomir; Horacek, Jan; Dlouhy, Tomas; Dostal, Vaclav (June 1, 2018). "Approximation of the economy of fusion energy". Energy. 152: 489–497. Bibcode:2018Ene...152..489E. doi:10.1016/j.energy.2018.03.130. ISSN 0360-5442.
- ^ "Levelized Cost of Energy and Levelized Cost of Storage 2019". Lazard.com. Archived from the original on 2023-02-19. Retrieved 2021-06-01.
- ^ Griffiths, Thomas; Pearson, Richard; Bluck, Michael; Takeda, Shutaro (October 1, 2022). "The commercialisation of fusion for the energy market: a review of socio-economic studies". Progress in Energy. 4 (4): 042008. Bibcode:2022PrEne...4d2008G. doi:10.1088/2516-1083/ac84bf. ISSN 2516-1083. S2CID 251145811.
- ^ Kembleton, R.; Bustreo, C. (2022). "Prospective research and development for fusion commercialisation". Fusion Engineering and Design. 178: 113069. Bibcode:2022FusED.17813069K. doi:10.1016/j.fusengdes.2022.113069. ISSN 0920-3796. S2CID 247338079.
- ^ Otake, Tomoko (April 14, 2023). "Japan adopts national strategy on nuclear fusion as competition intensifies". The Japan Times. Retrieved 2023-04-19.
- ^ Holland, Andrew (November 13, 2020). "Political and commercial prospects for inertial fusion energy". Philosophical Transactions of the Royal Society A: Mathematical, Physical and Engineering Sciences. 378 (2184): 20200008. Bibcode:2020RSPTA.37800008H. doi:10.1098/rsta.2020.0008. PMID 33040662. S2CID 222277887.
- ^ a b "Safety in Fusion". www.iaea.org. May 28, 2021. Retrieved 2021-06-01.
- ^ Slesinski, Daniel (January 28, 2021). "NRC Hosts Virtual Public Meeting on Developing Options for a Regulatory Framework for Fusion Energy". Fusion Industry Assn. Retrieved 2021-02-14.
- ^ Slesinski, Daniel (March 30, 2021). "NRC Hosts Second Virtual Public Meeting on Developing a Regulatory Framework for Fusion Energy". Fusion Industry Assn. Retrieved 2021-04-10.
- ^ Holland, Andrew (January 5, 2021). "Fusion Legislation Signed into Law". Fusion Industry Assn. Retrieved 2021-02-14.
- ^ Windridge, Melanie. "U.K Serious About Fusion: New Report On Regulation Recommends Proportionate, Agile Approach". Forbes. Retrieved 2021-06-03.
- ^ Holland, Andrew (June 1, 2021). "UK Regulatory Horizons Council Issues Report on Fusion Energy Regulation". Fusion Industry Assn. Archived from the original on 2023-04-20. Retrieved 2021-06-21.
- ^ Towards Fusion Energy: The UK Government's Fusion Strategy (PDF). London, UK: UK Government, Department for Business, Energy, & Industrial Strategy. 2021.
- ^ "Government sets out vision for UK's rollout of commercial fusion energy". GOV.UK. Retrieved 2021-10-15.
- ^ "UK government publishes fusion strategy – Nuclear Engineering International". www.neimagazine.com. October 5, 2021. Retrieved 2021-10-15.
- ^ Holland, Andrew (April 14, 2023). "NRC Decision Separates Fusion Energy Regulation from Nuclear Fission". Fusion Industry Assn. Retrieved 2023-04-19.
- ^ "New laws passed to bolster energy security and deliver net zero". GOV.UK. Retrieved 2023-11-10.
- ^ "Agile Nations: UK, Japan and Canada joint recommendations on fusion energy". GOV.UK. Retrieved 2024-03-20.
- ^ "Fusion Caucus Celebrates House Passage of Bipartisan Fusion Energy Act". U.S. Representative Don Beyer. February 29, 2024. Retrieved 2024-03-01.
- ^ a b Holland, Andrew. "Fusion energy needs smart federal government regulation". The Washington Times. Retrieved 2020-10-10.
- ^ Turrell, Arthur (August 28, 2021). "The race to give nuclear fusion a role in the climate emergency". the Guardian. Retrieved 2022-02-15.
- ^ a b Clery, Daniel (2014). A piece of the sun: the quest for fusion energy. New York: Overlook Duckworth. ISBN 978-1468310412. OCLC 1128270426.
- ^ "Will China beat the world to nuclear fusion and clean energy?". China Blog. BBC News. April 18, 2018. Retrieved 2020-10-12.
- ^ a b Carayannis, Elias G.; Draper, John; Bhaneja, Balwant (October 2, 2020). "Towards Fusion Energy in the Industry 5.0 and Society 5.0 Context: Call for a Global Commission for Urgent Action on Fusion Energy". Journal of the Knowledge Economy. 12 (4): 1891–1904. doi:10.1007/s13132-020-00695-5. ISSN 1868-7873. S2CID 222109349.
- ^ Carayannis, Elias G.; Draper, John (April 22, 2021). "The place of peace in the ITER machine assembly launch: Thematic analysis of the political speeches in the world's largest science diplomacy experiment". Peace and Conflict: Journal of Peace Psychology. 27 (4): 665–668. doi:10.1037/pac0000559. ISSN 1532-7949. S2CID 235552703.
- ^ Gi, Keii; Sano, Fuminori; Akimoto, Keigo; Hiwatari, Ryoji; Tobita, Kenji (2020). "Potential contribution of fusion power generation to low-carbon development under the Paris Agreement and associated uncertainties". Energy Strategy Reviews. 27: 100432. Bibcode:2020EneSR..2700432G. doi:10.1016/j.esr.2019.100432.
- ^ Nicholas, T. E. G.; Davis, T. P.; Federici, F.; Leland, J.; Patel, B. S.; Vincent, C.; Ward, S. H. (2021). "Re-examining the role of nuclear fusion in a renewables-based energy mix". Energy Policy. 149: 112043. arXiv:2101.05727. Bibcode:2021EnPol.14912043N. doi:10.1016/j.enpol.2020.112043. ISSN 0301-4215. S2CID 230570595.
- ^ Carayannis, Elias; Draper, John; Crumpton, Charles (2022). "Reviewing fusion energy to address climate change by 2050". Journal of Energy and Development. 47 (1).
- ^ "National Academies calls for a fusion pilot plant". Bulletin of the Atomic Scientists. April 14, 2021. Retrieved 2021-04-15.
- ^ "US must make an infrastructure investment in fusion energy". Washington Examiner. July 13, 2021. Retrieved 2021-07-16.
- ^ "An aggressive market-driven model for US fusion power development". MIT News | Massachusetts Institute of Technology. February 24, 2021. Retrieved 2021-02-26.
- ^ Cho, Adrian (February 19, 2021). "Road map to U.S. fusion power plant comes into clearer focus – sort of". Science. Retrieved 2021-03-06.
- ^ Kramer, David (March 10, 2021). "Academies urge public–private effort to build a pilot fusion-power plant". Physics Today. 2021 (2): 0310a. Bibcode:2021PhT..2021b.310.. doi:10.1063/PT.6.2.20210310a. S2CID 243296520.
- ^ "FIA Congratulates Congressional Bipartisan Fusion Caucus". Fusion Industry Association. February 19, 2021. Retrieved 2021-02-26.
- ^ Vries, Gieljan de (December 15, 2020). "Expert panel approves next DEMO design phase". www.euro-fusion.org. Retrieved 2021-02-16.
- ^ "At COP28, John Kerry unveils nuclear fusion strategy as a source of clean energy". AP News. December 5, 2023. Retrieved 2023-12-08.
- ^ Renshaw, Jarrett; Gardner, Timothy (April 10, 2024). "US, Japan announce partnership to accelerate nuclear fusion". Reuters.
- ^ Caroline (April 30, 2024). "G7 Puts Fusion Forward At The Climate, Energy And Environment Ministers' Meeting". Fusion Industry Association. Retrieved 2024-05-11.
- ^ "UK and Canada team up to solve nuclear fusion fuel shortage". Science|Business. Retrieved 2024-05-11.
- ^ Heeter, Robert F.; et al. "Conventional Fusion FAQ Section 2/11 (Energy) Part 2/5 (Environmental)". Fused.web.llnl.gov. Archived from the original on 2001-03-03. Retrieved 2014-10-30.
- ^ Stadermann, Frank J. "Relative Abundances of Stable Isotopes". Laboratory for Space Sciences, Washington University in St. Louis. Archived from the original on 2011-07-20.
- ^ Ongena, J.; Van Oost, G. "Energy for Future Centuries" (PDF). Laboratorium voor Plasmafysica – Laboratoire de Physique des Plasmas Koninklijke Militaire School – École Royale Militaire; Laboratorium voor Natuurkunde, Universiteit Gent. pp. Section III.B. and Table VI. Archived from the original (PDF) on 2011-07-27.
- ^ EPS Executive Committee. "The importance of European fusion energy research". The European Physical Society. Archived from the original on 2008-10-08.
- ^ "Space propulsion | Have fusion, will travel". ITER. Retrieved 2021-06-21.
- ^ Holland, Andrew (June 15, 2021). "Funding for Fusion for Space Propulsion". Fusion Industry Assn. Archived from the original on 2023-04-20. Retrieved 2021-06-21.
- ^ Schulze, Norman R.; United States; National Aeronautics and Space Administration; Scientific and Technical Information Program (1991). Fusion energy for space missions in the 21st century. Washington, DC; Springfield, Va.: National Aeronautics and Space Administration, Office of Management, Scientific and Technical Information Program; [For sale by the National Technical Information Service [distributor. OCLC 27134218.
- ^ "Principles of Fusion Energy Utilization in Space Propulsion". Fusion Energy in Space Propulsion. Progress in Astronautics and Aeronautics. American Institute of Aeronautics and Astronautics. January 1, 1995. pp. 1–46. doi:10.2514/5.9781600866357.0001.0046. ISBN 978-1563471841. Retrieved 2020-10-11.
- ^ Donn, Jeff (June 21, 2011). "Radioactive tritium leaks found at 48 US nuke sites". NBC News. Archived from the original on 2020-11-11. Retrieved 2023-07-04.
- ^ Abdou, M.; et al. (2020). "Physics and technology considerations for the deuterium-tritium fuel cycle and conditions for tritium fuel self sufficiency". Nuclear Fusion. 61 (1): 013001. doi:10.1088/1741-4326/abbf35. S2CID 229444533.
- ^ Stix, T. H. (1998). "Highlights in early stellarator research at Princeton". Helical System Research.
- ^ Johnson, John L. (November 16, 2001). The Evolution of Stellarator Theory at Princeton (Technical report). doi:10.2172/792587. OSTI 792587.
- ^ Irvine, Maxwell (2014). Nuclear power: a very short introduction. Oxford: Oxford University Press. ISBN 978-0199584970. OCLC 920881367.
- ^ Kusama, Y. (2002), Stott, Peter E.; Wootton, Alan; Gorini, Giuseppe; Sindoni, Elio (eds.), "Requirements for Diagnostics in Controlling Advanced Tokamak Modes", Advanced Diagnostics for Magnetic and Inertial Fusion, Boston, MA: Springer US, pp. 31–38, doi:10.1007/978-1-4419-8696-2_5, ISBN 978-1441986962
- ^ Menard, J. E. (February 4, 2019). "Compact steady-state tokamak performance dependence on magnet and core physics limits". Philosophical Transactions of the Royal Society A: Mathematical, Physical and Engineering Sciences. 377 (2141): 20170440. Bibcode:2019RSPTA.37770440M. doi:10.1098/rsta.2017.0440. ISSN 1364-503X. PMC 6365855. PMID 30967044.
- ^ Kaw, P. K. (1999). "Steady state operation of tokamaks". Nuclear Fusion. 39 (11): 1605–1607. doi:10.1088/0029-5515/39/11/411. ISSN 0029-5515. S2CID 250826481.
- ^ Key, M.H. (1985). "Highlights of laser fusion related research by United Kingdom universities using the SERC Central Laser Facility at the Rutherford Appleton Laboratory". Nuclear Fusion. 25 (9): 1351–1353. doi:10.1088/0029-5515/25/9/063. S2CID 119922168.
- ^ Verlarde, G.; Carpintero–Santamaría, Natividad, eds. (2007). Inertial confinement nuclear fusion : a historical approach by its pioneers. London: Foxwell & Davies (UK). ISBN 978-1905868100. OCLC 153575814.
- ^ McKinzie, Matthew; Paine, Christopher E. (2000). "When peer review fails: The Roots of the National Ignition Facility (NIF) Debacle". National Resources Defense Council. Retrieved 2014-10-30.
- ^ "Tore Supra". Archived from the original on 2012-11-15. Retrieved 2016-02-03.
- ^ Smirnov, V. P. (December 30, 2009). "Tokamak foundation in USSR/Russia 1950–1990" (PDF). Nuclear Fusion. 50 (1): 014003. doi:10.1088/0029-5515/50/1/014003. ISSN 0029-5515. S2CID 17487157.
- ^ Y-K Martin Peng, "Spherical Torus, Compact Fusion at Low Yield". Oak Ridge National Laboratory/FEDC-87/7 (December 1984)
- ^ a b Sykes, Alan (1997). "High β produced by neutral beam injection in the START (Small Tight Aspect Ratio Tokamak) spherical tokamak". Physics of Plasmas. 4 (5): 1665–1671. Bibcode:1997PhPl....4.1665S. doi:10.1063/1.872271. ISSN 1070-664X.
- ^ Braams, C. M.; Stott, P. E. (2002). Nuclear fusion: half a century of magnetic confinement fusion research. Institute of Physics Pub. ISBN 978-0367801519. OCLC 1107880260.
- ^ Jarvis, O. N. (June 16, 2006). "Neutron measurements from the preliminary tritium experiment at JET (invited)". Review of Scientific Instruments. 63 (10): 4511–4516. doi:10.1063/1.1143707.
- ^ Garin, Pascal (October 2001). "Actively cooled plasma facing components in Tore Supra". Fusion Engineering and Design. 56–57: 117–123. Bibcode:2001FusED..56..117G. doi:10.1016/s0920-3796(01)00242-3. ISSN 0920-3796.
- ^ European Commission Directorate-General for Research and Innovation (2004). Fusion Research: An Energy Option for Europe's Future. Luxembourg: Office for Official Publications of the European Communities. ISBN 92-894-7714-8. OCLC 450075815.
- ^ Claessens, Michel (2020). ITER: The Giant Fusion Reactor. doi:10.1007/978-3-030-27581-5. ISBN 978-3030275808. S2CID 243590344.
- ^ Atzeni, Stefano (2004). The physics of inertial fusion : beam plasma interaction, hydrodynamics, hot dense matter. Meyer-ter-Vehn, Jürgen. Oxford: Clarendon Press. ISBN 978-0198562641. OCLC 56645784.
- ^ Pfalzner, Susanne (March 2, 2006). An Introduction to Inertial Confinement Fusion. CRC Press. doi:10.1201/9781420011845. ISBN 978-0429148156.
- ^ "People's Daily Online – China to build world's first "artificial sun" experimental device". en.people.cn. Archived from the original on 2011-06-05. Retrieved 2020-10-10.
- ^ "What Is the National Ignition Facility?". lasers.llnl.gov. Lawrence Livermore National Laboratory. Archived from the original on 2017-07-31. Retrieved 2022-08-07.
- ^ Kanellos, Michael. "Hollywood, Silicon Valley and Russia Join Forces on Nuclear Fusion". Forbes. Retrieved 2017-08-21.
- ^ Frochtzwajg, Jonathan. "The secretive, billionaire-backed plans to harness fusion". BBC. Retrieved 2017-08-21.
- ^ Clery, Daniel (July 25, 2014). "Fusion's restless pioneers". Science. 345 (6195): 370–375. Bibcode:2014Sci...345..370C. doi:10.1126/science.345.6195.370. ISSN 0036-8075. PMID 25061186.
- ^ Gray, Richard (April 19, 2017). "The British reality TV star building a fusion reactor". BBC. Retrieved 2017-08-21.
- ^ Clery, Daniel (April 28, 2017). "Private fusion machines aim to beat massive global effort". Science. 356 (6336): 360–361. Bibcode:2017Sci...356..360C. doi:10.1126/science.356.6336.360. ISSN 0036-8075. PMID 28450588. S2CID 206621512.
- ^ SPIE Europe Ltd. "PW 2012: fusion laser on track for 2012 burn". Optics.org. Retrieved 2013-06-22.
- ^ "Nuclear fusion milestone passed at US lab". BBC News. Retrieved 2014-10-30.
- ^ "The Alectryon High Yield Neutron Generator". Phoenix Nuclear Labs. 2013.
- ^ Chandler, David L. (August 10, 2015). "A small, modular, efficient fusion plant". MIT News. MIT News Office.
- ^ Sunn Pedersen, T.; Andreeva, T.; Bosch, H. -S; Bozhenkov, S.; Effenberg, F.; Endler, M.; Feng, Y.; Gates, D. A.; Geiger, J.; Hartmann, D.; Hölbe, H.; Jakubowski, M.; König, R.; Laqua, H. P.; Lazerson, S.; Otte, M.; Preynas, M.; Schmitz, O.; Stange, T.; Turkin, Y. (November 2015). "Plans for the first plasma operation of Wendelstein 7-X". Nuclear Fusion. 55 (12): 126001. Bibcode:2015NucFu..55l6001P. doi:10.1088/0029-5515/55/12/126001. hdl:11858/00-001M-0000-0029-04EB-D. S2CID 67798335.
- ^ Pedersen, T. Sunn; Otte, M.; Lazerson, S.; Helander, P.; Bozhenkov, S.; Biedermann, C.; Klinger, T.; Wolf, R. C.; Bosch, H. -S.; Abramovic, Ivana; Äkäslompolo, Simppa; Aleynikov, Pavel; Aleynikova, Ksenia; Ali, Adnan; Alonso, Arturo; Anda, Gabor; Andreeva, Tamara; Ascasibar, Enrique; Baldzuhn, Jürgen; Banduch, Martin; Barbui, Tullio; Beidler, Craig; Benndorf, Andree; Beurskens, Marc; Biel, Wolfgang; Birus, Dietrich; Blackwell, Boyd; Blanco, Emilio; Blatzheim, Marko; et al. (2016). "Confirmation of the topology of the Wendelstein 7-X magnetic field to better than 1:100,000". Nature Communications. 7: 13493. Bibcode:2016NatCo...713493P. doi:10.1038/ncomms13493. PMC 5141350. PMID 27901043.
- ^ Wolf, R. C.; Alonso, A.; Äkäslompolo, S.; Baldzuhn, J.; Beurskens, M.; Beidler, C. D.; Biedermann, C.; Bosch, H.-S.; Bozhenkov, S.; Brakel, R.; Braune, H.; Brezinsek, S.; Brunner, K.-J.; Damm, H.; Dinklage, A.; Drewelow, P.; Effenberg, F.; Feng, Y.; Ford, O.; Fuchert, G.; Gao, Y.; Geiger, J.; Grulke, O.; Harder, N.; Hartmann, D.; Helander, P.; Heinemann, B.; Hirsch, M.; Höfel, U.; Hopf, C.; Ida, K.; Isobe, M.; Jakubowski, M. W.; Kazakov, Y. O.; Killer, C.; Klinger, T.; Knauer, J.; König, R.; Krychowiak, M.; Langenberg, A.; Laqua, H. P.; Lazerson, S.; McNeely, P.; Marsen, S.; Marushchenko, N.; Nocentini, R.; Ogawa, K.; Orozco, G.; Osakabe, M.; Otte, M.; Pablant, N.; Pasch, E.; Pavone, A.; Porkolab, M.; Puig Sitjes, A.; Rahbarnia, K.; Riedl, R.; Rust, N.; Scott, E.; Schilling, J.; Schroeder, R.; Stange, T.; von Stechow, A.; Strumberger, E.; Sunn Pedersen, T.; Svensson, J.; Thomson, H.; Turkin, Y.; Vano, L.; Wauters, T.; Wurden, G.; Yoshinuma, M.; Zanini, M.; Zhang, D. (August 1, 2019). "Performance of Wendelstein 7-X stellarator plasmas during the first divertor operation phase". Physics of Plasmas. 26 (8): 082504. Bibcode:2019PhPl...26h2504W. doi:10.1063/1.5098761. hdl:1721.1/130063. S2CID 202127809.
- ^ Sunn Pedersen, Thomas; et al. (April 2022). "Experimental confirmation of efficient island divertor operation and successful neoclassical transport optimization in Wendelstein 7-X". Nuclear Fusion. 62 (4): 042022. Bibcode:2022NucFu..62d2022S. doi:10.1088/1741-4326/ac2cf5. hdl:1721.1/147631. S2CID 234338848.
- ^ Max Planck Institute for Experimental Physics (February 3, 2016). "Wendelstein 7-X fusion device produces its first hydrogen plasma". www.ipp.mpg.de. Retrieved 2021-06-15.
- ^ Wang, Brian (August 1, 2018). "Nuclear Fusion Updated project reviews". www.nextbigfuture.com. Retrieved 2018-08-03.
- ^ MacDonald, Fiona (May 2017). "The UK Just Switched on an Ambitious Fusion Reactor – And It Works". ScienceAlert. Retrieved 2019-07-03.
- ^ "Italy's Eni defies sceptics, may up stake in nuclear fusion project". Reuters. April 13, 2018.
- ^ "MIT Aims to Harness Fusion Power Within 15 years". April 3, 2018.
- ^ "MIT Aims To Bring Nuclear Fusion To The Market In 10 Years". March 9, 2018.
- ^ Chandler, David (March 9, 2018). "MIT and newly formed company launch novel approach to fusion power". MIT News. Massachusetts Institute of Technology.
- ^ Molodyk, A.; Samoilenkov, S.; Markelov, A.; Degtyarenko, P.; Lee, S.; Petrykin, V.; Gaifullin, M.; Mankevich, A.; Vavilov, A.; Sorbom, B.; Cheng, J.; Garberg, S.; Kesler, L.; Hartwig, Z.; Gavrilkin, S.; Tsvetkov, A.; Okada, T.; Awaji, S.; Abraimov, D.; Francis, A.; Bradford, G.; Larbalestier, D.; Senatore, C.; Bonura, M.; Pantoja, A. E.; Wimbush, S. C.; Strickland, N. M.; Vasiliev, A. (January 22, 2021). "Development and large volume production of extremely high current density YBa 2 Cu 3 O 7 superconducting wires for fusion". Scientific Reports. 11 (1): 2084. doi:10.1038/s41598-021-81559-z. PMC 7822827. PMID 33483553.
- ^ Clery, Daniel (April 8, 2021). "With "smoke ring" technology, fusion startup marks steady progress". Science | AAAS. Retrieved 2021-04-11.
- ^ Morris, Ben (September 30, 2021). "Clean energy from the fastest moving objects on earth". BBC News. Retrieved 2021-12-09.
- ^ Session AR01: Review: Creating A Burning Plasma on the National Ignition Facility. 63rd Annual Meeting of the APS Division of Plasma Physics, November 8–12, 2021; Pittsburgh, PA. Bulletin of the American Physical Society. Vol. 66, no. 13.
- ^ a b c d Wright, Katherine (November 30, 2021). "Ignition First in a Fusion Reaction". Physics. 14: 168. Bibcode:2021PhyOJ..14..168W. doi:10.1103/Physics.14.168. S2CID 244829710.
- ^ Dunning, Hayley (August 17, 2021). "Major nuclear fusion milestone reached as "ignition" triggered in a lab". Science X Network.
- ^ Bishop, Breanna (August 18, 2021). "National Ignition Facility experiment puts researchers at threshold of fusion ignition". Lawrence Livermore National Laboratory.
- ^ Conca, James. "Helion Energy Raises $500 Million On The Fusion Power Of Stars". Forbes. Retrieved 2021-12-19.
- ^ Journal, Jennifer Hiller | Photographs by Tony Luong for The Wall Street (December 1, 2021). "WSJ News Exclusive | Nuclear-Fusion Startup Lands $1.8 Billion as Investors Chase Star Power". Wall Street Journal. ISSN 0099-9660. Retrieved 2021-12-17.
- ^ Blain, Loz (April 6, 2022). "Oxford spinoff demonstrates world-first hypersonic "projectile fusion"". New Atlas. Retrieved 2022-04-06.
- ^ a b c Osaka, Shannon (December 12, 2022). "What you need to know about the U.S. fusion energy breakthrough". The Washington Post. Retrieved 2022-12-13.
- ^ Hartsfield, Tom (December 13, 2022). "There is no "breakthrough": NIF fusion power still consumes 130 times more energy than it creates". Big Think.
- ^ Gardner, Timothy (June 1, 2023). "US announces $46 million in funds to eight nuclear fusion companies". Reuters.
- ^ Dobberstein, Laura (December 4, 2023). "World's largest nuclear fusion reactor comes online in Japan". The Register. Situation Publishing.
- ^ "S. Korea's artificial sun project KSTAR achieves longest operation time of 102 seconds". Aju Business Daily. March 21, 2024.
- ^ Lerner, Eric J.; Murali, S. Krupakar; Shannon, Derek; Blake, Aaron M.; Van Roessel, Fred (March 23, 2012). "Fusion reactions from >150 keV ions in a dense plasma focus plasmoid". Physics of Plasmas. 19 (3): 032704. Bibcode:2012PhPl...19c2704L. doi:10.1063/1.3694746. S2CID 120207711.
- ^ Halper, Mark (March 28, 2012). "Fusion breakthrough". Smart PLanet. Retrieved 2012-04-01.
- ^ "JET". Culham Centre Fusion Energy. Archived from the original on 2016-07-07. Retrieved 2016-06-26.
- ^ Tischler, Karl (February 8, 2024). "Breaking New Ground: JET Tokamak's Latest Fusion Energy Record Shows Mastery of Fusion Processes". EUROfusion. Retrieved 2024-02-11.
- ^ Obenschain, Stephen; et al. (2015). "High-energy krypton fluoride lasers for inertial fusion". Applied Optics. 54 (31): F103–F122. Bibcode:2015ApOpt..54F.103O. doi:10.1364/AO.54.00F103. PMID 26560597.
- ^ "Krypton Fluoride (KrF) Laser Driver for Inertial Fusion Energy"
- ^ "New record for fusion". MIT News | Massachusetts Institute of Technology. October 14, 2016. Retrieved 2020-10-11.
- ^ "World Highest Fusion Triple Product Marked in High-βp H-mode Plasmas". Archived from the original on 2013-01-06.
- ^ "Measuring Progress in Fusion Energy: The Triple Product". www.fusionenergybase.com. Archived from the original on 2020-10-01. Retrieved 2020-10-10.
- ^ Cohen, Sam, and B. Berlinger. "Long-pulse Operation of the PFRC-2 Device." The Joint US-Japan Compact Torus. Wisconsin, Madison. 22 Aug. 2016. Lecture.
- ^ "Successful second round of experiments with Wendelstein 7-X". www.ipp.mpg.de. Retrieved 2019-03-22.
- ^ Lavars, Nick (November 26, 2018). "Wendelstein 7-X fusion reactor keeps its cool en route to record-breaking results". newatlas.com. Retrieved 2018-12-01.
- ^ Magazine, Smithsonian; Gamillo, Elizabeth. "China's Artificial Sun Just Broke a Record for Longest Sustained Nuclear Fusion". Smithsonian Magazine.
- ^ "China's "Artificial Sun" Fusion Reactor Just Set a World Record". Futurism. June 2, 2021.
- ^ Alan Sykes, "The Development of the Spherical Tokamak" Archived July 22, 2011, at the Wayback Machine, ICPP, Fukuoka September 2008
- ^ Szondy, David (March 13, 2022). "Tokamak Energy achieves temperature threshold for commercial fusion". New Atlas. Retrieved 2022-03-15.
- ^ Lavars, Nick (November 24, 2021). "KSTAR fusion reactor sets record with 30-second plasma confinement". New Atlas. Retrieved 2022-03-15.
Bibliography[edit]
- Clery, Daniel (2014). A Piece of the Sun: The Quest for Fusion Energy. The Overlook Press. ISBN 978-1468310412.
- Cockburn, Stewart; Ellyard, David (1981). Oliphant, the life and times of Sir Mark Oliphant. Axiom Books. ISBN 978-0959416404.
- Dean, Stephen O. (2013). Search for the Ultimate Energy Source: A History of the U.S. Fusion Energy Program. Springer Science & Business Media. ISBN 978-1461460374.
- Hagelstein, Peter L.; McKubre, Michael; Nagel, David; Chubb, Talbot; Hekman, Randall (2004). "New Physical Effects in Metal Deuterides" (PDF). 11th Condensed Matter Nuclear Science. Vol. 11. Washington: US Department of Energy. pp. 23–59. Bibcode:2006cmns...11...23H. CiteSeerX 10.1.1.233.5518. doi:10.1142/9789812774354_0003. ISBN 978-9812566409. Archived from the original (PDF) on 2007-01-06. (manuscript)
- Hutchinson, Alex (January 8, 2006). "The Year in Science: Physics". Discover Magazine (Online). ISSN 0274-7529. Retrieved 2008-06-20.
- Nuttall, William J., Konishi, Satoshi, Takeda, Shutaro, and Webbe-Wood, David (2020). Commercialising Fusion Energy: How Small Businesses are Transforming Big Science. IOP Publishing. ISBN 978-0750327176.
- Molina, Andrés de Bustos (2013). Kinetic Simulations of Ion Transport in Fusion Devices. Springer International Publishing. ISBN 978-3319004211.
- Nagamine, Kanetada (2003). "Muon Catalyzed Fusion". Introductory Muon Science. Cambridge University Press. ISBN 978-0521038201.
- Pfalzner, Susanne (2006). An Introduction to Inertial Confinement Fusion. US: Taylor & Francis. ISBN 978-0750307017.
Further reading[edit]
- Ball, Philip. "The chase for fusion energy". Nature. Retrieved 2021-11-22.
- Oreskes, Naomi, "Fusion's False Promise: Despite a recent advance, nuclear fusion is not the solution to the climate crisis", Scientific American, vol. 328, no. 6 (June 2023), p. 86.